Computed Tomography Physics
In the past decade, computed tomography (CT) has undergone tremendous technical advances. In 1992, the first dual-slice CT scanner (CT Twin, formerly Elscint Technologies, Haifa, Israel) was introduced. In 1998, the quad-slice CT scanners were introduced. In 2002, the 16-slice CT scanner became available. With these scanners it became possible to perform coronary CT angiography, multiphasic examinations, and virtual endoscopy studies. In 2004, 64-slice CT scanners were introduced. With 64-slice CT scanners it became possible to acquire submillimeter sections of large body regions, perform high-quality coronary angiography in most patients, and perform perfusion studies.
Dual-Energy Computed Tomography
The evolving modality of dual-energy computed tomography (DECT) has specific capacities beyond single-energy CT that can translate into improved abdominal imaging. DECT consists of acquisition of two different image data sets with different kilovoltage peaks, usually 80 and 140 kVp, during the same study. It was first described in 1976, but its clinical applications became possible only recently with the introduction of multidetector computed tomography (MDCT). Compton scatter and the photoelectric effect are the two primary ways that the x-ray interacts with the materials. The probability of an x-ray undergoing photoelectric effect increases in substances with higher atomic number and at lower energy levels, giving higher attenuation. For example, iodine ( Z = 53) has higher attenuation than calcium ( Z = 20), which is in turn more attenuating than water.
X-ray attenuation properties at low- and high-energy acquisitions are also used to differentiate materials by mathematically transforming attenuation measurements of an object at low and high kVp as a function of two materials that would be needed to represent the measured attenuation. Besides being used to characterize the composition of the tissue, this information can be mathematically manipulated to generate postprocessed images that can simulate unenhanced data sets, images with the capability to quantify iodine in the tissues, and also virtual images simulating monochromatic energies. In addition, blended images with desired contributions from acquisitions that are of both low and high kilovoltage peak can be processed. By appropriately blending high- and low-energy series, images analogous to those obtained with single-energy 120-kVp acquisition can be obtained.
The dual-source (dsDECT) system consists of two x-ray tubes that acquire data with different energies (low and high kVp) in a DE acquisition. The single-source system (ssDECT) uses a single x-ray tube, which can alternate tube energy (low and high kVp) in less than 0.5 ms, in the same gantry rotation. The single source sandwich detector (sssdDECT) uses a detector with two layers that separate high- and low-energy photons from a single x-ray source from which two image data sets are generated.
The dsDECT approach for material separation uses the three-material decomposition method, which takes into account known x-ray absorption properties of three materials (iodine, soft tissue, and fat) at dual-energy acquisition. Based on different attenuations at a specific location in the CT acquisition at two different tube potentials, the expected amount of each material (selected for the decomposition) is calculated and the density of this point is determined. This process generates iodine images and virtual nonenhanced images (similar to a nonenhanced acquisition).
On ssDECT, by using the material decomposition process for two known selected basis materials, the attenuation measurements from high- and low-energy data can be mathematically transformed into the density (or amount) of the two known materials that would be needed to produce the kilovoltage peaks of low and high attenuation. Usually, water and iodine (a low- and a high-attenuating material, respectively) are selected, resulting in material density pairing—iodine images and water images (latter being similar to a nonenhanced acquisition).
From both DE systems, virtual monochromatic images at desired energy level can be generated through a complex mathematical calculation, enabling the representation of the data as though it came from a monochromatic single energy source.
Pitch, Noise, and Radiation Dose
In a single-slice scanner, the pitch of the helical scan refers to the ratio of the table movement per gantry rotation to the slice width. This definition is not entirely satisfactory when applied to multislice CT scanners. A better definition of pitch in multislice CT is the ratio of table movement to the total x-ray beam collimation. Using this definition, the lengths of volume scanned by 64-slice CT scanners, when a pitch of 1 is chosen, range from 58 to 114 mm.
In single-slice CT, the gantry always has to rotate through a specific angle, depending on the reconstruction algorithm used, to acquire the projection information needed to create an axial image. Thus, the number of photons that are used to produce an axial image (the principal determinant of image noise) depends on the tube current/time product and not on the pitch. However, as the pitch increases, the radiation dose reduces, because each part of the scanned volume spends less time in the x-ray beam.
The relationship among pitch, noise, and radiation dose is not the same with current multislice scanners. In the noncardiac mode, as the pitch is increased, 64-slice scanners automatically increase the tube current to maintain a relatively constant noise level. With higher pitch, the larger focal spot may be automatically chosen. These secondary effects negate any reduction of radiation dose that is intuitively expected with an increase in pitch. Therefore, changing the pitch in current multislice CT scanners does not necessarily alter the radiation dose.
Reducing Radiation Dose
CT examination is responsible for approximately 70% of the radiation dose received by the general population from imaging. Modern scanners employ techniques to reduce radiation dose. Automatic tube current modulation (ATCM) changes the tube current to produce an acceptable noise level for each slice. The tube current modulation may be in the radial (x-y) plane ( Figure 6-1, A ) or in the z-axis (see Figure 6-1, B ). Although the modulation techniques work differently in each scanner, in general the milliampere level is altered to maintain a selected noise level throughout the scanned volume. In some scanners, such as Sensation 64 (Siemens Medical Solutions, Malvern, PA), the tube current is constantly varied using dosimetry readings from prior gantry rotations. In others, the localizer image is used to calculate regions requiring higher and lower x-ray tube currents. It is possible on most 64-slice scanners to use simultaneously both radial and z-axis ATCM, leading to an overall reduction in dose of 40% to 60% compared with fixed milliampere scanning. The use of ATCM has been shown to maintain image quality in assessment of structures with high inherent contrast differences, such as in chest CT and CT colonography. However, there is a potential of ATCM to increase noise and adversely affect image quality where the difference in tissue contrast is low.
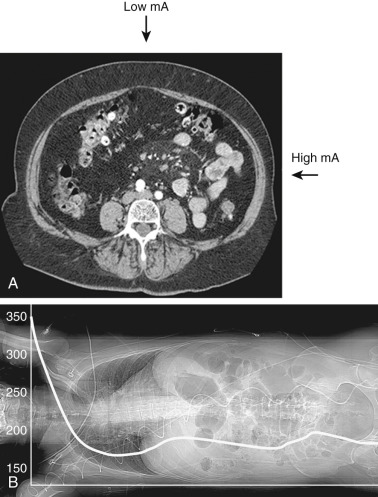
Artifacts of Multislice Scanners
Artifacts caused by beam hardening, photon starvation, and metallic implants occur less commonly in 40- and 64-channel scanners than they did in earlier CT generations. The use of beam-hardening reduction software, adaptive filtration, and ATCM reduces artifacts from dense bone such as in the shoulders and posterior cranial fossa. Adaptive filtration refers to software that smooths the attenuation differences at sites of highly variable attenuation, before reconstruction of source images. Several methods are now available to reduce artifacts from metallic implants, allowing diagnosis of prosthetic malfunction using CT. The use of thin-slice isotropic scanning technique almost completely eliminates stair-step artifacts seen on multiplanar reformatted images. Ring artifacts may be seen as a result of poorly functional or incorrectly calibrated detector elements ( Figure 6-2 ).
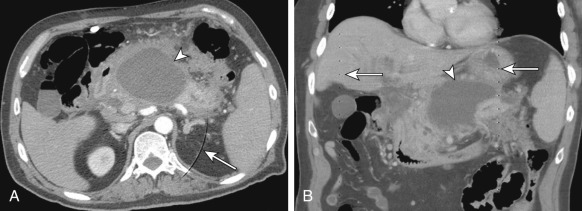
The wide cone beam required for 40- and 64-slice scanning as well the obligatory reconstruction algorithms result in specific artifacts. These include cone beam, windmill, and zebra artifacts.
Cone Beam Artifact
Cone beam artifacts result from approximations in reconstruction algorithms that fail to exactly reconstruct a widely divergent x-ray beam. The artifact may manifest as geometric distortions or a glow around high-contrast objects such as bones ( Figure 6-3 ). The widely divergent cone beam of 64-slice scanners would be expected to show worse cone beam artifact than narrower beams of 4- and 16-slice scanners. However, 64-slice scanners use three-dimensional reconstruction techniques that minimize this artifact.
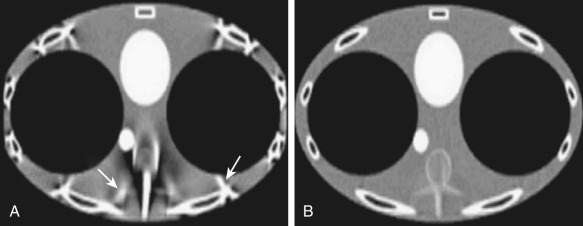
Windmill Artifact
Windmill or splay artifacts occur as a result of inadequate sampling in the z-direction ( Figure 6-4 ). These artifacts are similar to aliasing artifacts seen in Doppler ultrasonography and are unrelated to the cone beam geometry. The appearance of artifacts is determined by the number of detector rows, the detector width, the helical pitch, and the reconstructed slice thickness. For example, splay is more prominent on thin-slice axial images in which the slice thickness is approximately equal to the detector width and they are minimized as the slice thickness is increased. Typically, splay artifact does not occur if the slice thickness is double that of the detector width. The number of vanes in the windmill artifact is proportional to the pitch; decreasing the pitch also reduces this artifact.
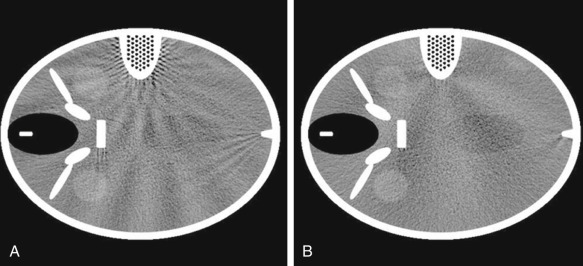
Zebra Artifact
Weighting factors used in reconstruction algorithms are associated with inhomogeneity in noise, particularly away from the center of rotation. The variation in noise between different axial slices becomes obvious on images oriented in the z-axis, including nonaxial reformatted images and maximum intensity projection images. The artifact appears as faint stripes of variable density and is termed the zebra artifact ( Figure 6-5 ). This artifact is reduced by ATCM techniques that attempt to maintain a constant noise level at different axial locations. Adaptive filtration techniques also can be used to reduce variability of noise between different axial slices. Zebra artifact is not related to beam hardening or the effect of scatter radiation, which may cause a similar-appearing artifact on nonhelical scanners.
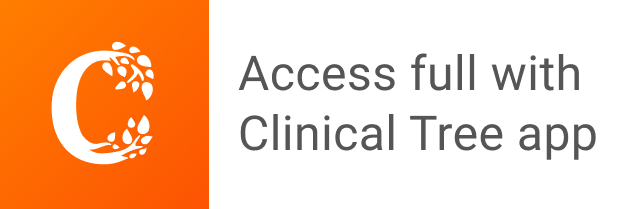