Introduction
Radiotherapy is used as one of the major treatment modalities for patients with malignant diseases at different disease stages. Currently, the most common radiation choice for the majority of cancers is photon (x-ray)-based intensity-modulated external beam radiotherapy. Notably, recent advances in technology and basic and clinical research have facilitated the safe delivery of more effective and noninvasive radiotherapy for malignant diseases using charged particles, including intensity-modulated proton therapy. Proton beams deliver most of their energy at the distal edge of their range (the Bragg peak), which leads to an increase of the radiation doses to the clinical targets and minimization of the irradiation dose to adjacent normal tissues. Moreover, photon beams are categorized as low linear energy transfer (LET), whereas proton beams, especially in the spread-out Bragg peak (SOBP), which majorly contains Bragg peaks, are categorized as high LET. Thus in addition to the physical dose distribution advantage, proton therapy also presents distinct biology advantages compared with photon radiation. Even though the biological features of tumors and normal tissues after photon radiation have been extensively studied, the biological responses of tumors and normal tissues to proton radiation are far from clear. The biological properties of proton beams that differ significantly from those of photons will be summarized in this chapter. Particular emphasis is placed on relative biological effectiveness (RBE), DNA damage, and repair effects induced by protons, proton beam–induced cell death mechanisms, the impact of proton beams on tumor immune responses, and the influence of proton beams on tumor angiogenesis.
DNA damage and repair
DNA damage
DNA is the critical target of radiation. Photon radiation induces DNA damage by the direct action of deposing beam energy to DNA. Photon radiation also induces DNA damage by the indirect action of forming reactive species near the DNA, primarily by turning a water molecule into a free radical (hydroxyl radicals, OH). Photon radiation causes many types of DNA damage, including single-base damage and single-strand breaks (isolated), and clustered base damage and double-strand breaks (DSBs) (clustered within a few DNA helical turns). Most single-strand breaks can be repaired normally. However, repair is more difficult, and erroneous rejoining of broken ends in DSBs may result in significant biological consequences. Failure of DNA DSBs repair results in induction of mutations, chromosome aberrations, cell death, or even possibly in malignant cell transformation. It is believed that the complexity of DNA damage is the determining factor for the consequent cellular response to radiation.
Although photon radiation–related DNA damage and repair researches have been conducted extensively, the DNA damage and repair as a consequence of proton radiation remain poorly understood. Similar to that of photon radiation, it has been proven that the indirect effect of proton beams plays a major role and causes a large proportion of DNA damage compared with the direct effect of proton beams. , However, Monte Carlo simulations have indicated that the average number of DNA damages per cluster tends to increase with the increasing of the radiation beam LET, which implies a higher level of DNA damage complexity induced by proton beams versus by that caused by photon beams. , These mathematical model prediction results have been verified by several other studies by testing DNA plasmids or cell lines. Using DNA plasmids pBR322 or T7 as testing material, the direct damage effect of proton beams to DNA was proven to generate more DSB clusters compared with non-DSB clusters compared with photon beams. , Similar observations were also demonstrated in cell-based studies. An increased complexity of DNA damages and slower DNA damage repair kinetics were observed in the human skin fibroblast AG01522 cells at the distal end of the SOBP after proton radiation. Other than this, large foci, which represent the DSB clusters, were also found more commonly in Chinese hamster ovary (CHO) cell lines CHO10B2 and irs-20 after proton radiation compared with photon radiation. The more severe DNA damage caused by proton beams were also proven by another study on the thyroid-stimulating hormone–dependent Fischer rat thyroid cells. The authors found more free-end DNAs 1 hour after proton radiation than photon radiation, which means a more rapid DNA damage repair in the cells exposed to photon beams than those exposed to proton beams. They further verified their results by finding a higher rate of micronucleus formation and the presence of larger micronuclei in cells treated by proton beams than those cells treated with photon beams. Persistent DNA damage was also observed in different head and neck cancer cell lines after exposure to proton beams versus exposure to photon beams. However, conflicting results were observed in a study using the DNA plasmid pBR322. In this study, the authors did not find a difference in the amount of the clustered DNA damage induced by proton beams compared with photon beams in either the liquid or in the dry samples.
DNA damage repair
Because DNA DSBs are the key lesion leading to severe biological consequences in cells exposed to photon radiation, it is meaningful to study DSB and its processing after cells are exposed to proton beams. Because the DNA damages induced by proton beams are with higher complexity than those induced by photon beams, the repair of the damaged DNA caused by proton beams may be different from the repair of damaged DNA caused by photon beams. Compared with the extensively studied DNA damage repair mechanisms after photon beam exposure, the study of DNA damage repair after proton beam radiation is limited, and mechanisms underlying the DNA damage repair are still to be uncovered.
There are two major distinguished DSB repair pathways ( Fig. 1.1 ): homologous recombination (HR) and nonhomologous end joining (NHEJ). NHEJ is active throughout the cell cycle and is the predominant repair pathway for photon radiation–induced DSBs in mammalian cells. , The roles of HR and NHEJ in DNA damage repair in response to proton versus photon beams have been studied. In this study, the CHO cells AA8, CHO9, UV5, Irs1sf, and XR-C1 with different Rad51 (a protein related to HR , ) and DNA-PKcs (a protein related to NHEJ , ) status were exposed to proton or photon beams. Cell survival and DSB repair were evaluated after radiation. The authors found that when compared with wild-type cells, Rad-51-deficient or suppressed cells have a higher proton versus photon radiation response rate; however, DNA-PKcs-deficient cells have not shown a different response rate to proton versus photon radiation when compared with wild-type cells. Moreover, delayed DSB repair was also found in the Rad-51-deficient cells after proton radiation. The authors concluded that HR is preferentially required for proton beam–induced DSB repair. A similar phenomenon was also found in human lung adenocarcinoma (A549) and human glioblastoma (M059K and M059J) cells. In this study, after blocking DNA-PKcs, a higher level of delayed DSBs repair and a more profound radio response was observed after cells were exposed to photon versus proton beams. On the other hand, depleting RAD51 led to an enhanced response of A549 cells to proton beams. The authors claimed a preference of HR versus NHEJ in proton beam–induced DSB repair. On the contrary, conflicting results were reported by others. In one study, the authors compared the DSB repair of the DNA-PKcs wild-type CHO cell line CHO10B2 with its derived radiosensitive mutant cell line, the DNA-PKcs-deficient cell line irs-20 after cells were exposed to photon and proton beams. Irs-20 cells presented more persistent DSBs compared with CHO10B cells after cells were exposed to both photon and proton beams. A dependence on the DNA-PKcs in repairing DSBs caused by both proton and photon beams was verified. In another study involving the DNA-PKcs wild-type CHO cell line CHO10B2, Ku80-mutated CHO mutant cell XRS-5, DNA-PKcs null V3 cells, Rad51D-mutated 51D1 cells, and 14 cell lines derived from V3 cells with complementary human DNA PKcs containing amino acid substitutions at specific positions, the cell responses to proton beams versus photon beams were not correlated with the status of DNA-PKcs or RAD51; thus, no preferential DSB repair pathway of HR or NHEJ was observed in proton beam–induced DSB repair. Other than the previously mentioned, a study using cervical cancer HeLa cells claimed that the higher cell response rate of proton beams versus photon beams in the SOBP is in an Artemis protein–dependent manner. Because Artemis protein is a member of the NHEJ pathway, this result reflects the dependency of the repair of proton beam–induced DSBs on NHEJ. Some other studies also demonstrated the preference of the NHEJ pathway in the repair of DSBs induced by proton beams. , This evidence includes the activation of Ataxia-Telangiesctasia mutated (ATM, contributing to NHEJ ) and DNA-PKcs but not ataxia telangiectasia and Rad3 related (ATR) by proton beams in human lung adenocarcinoma A549 cells and the induction of ATM by proton beams in human prostate cancer PC3 cells.
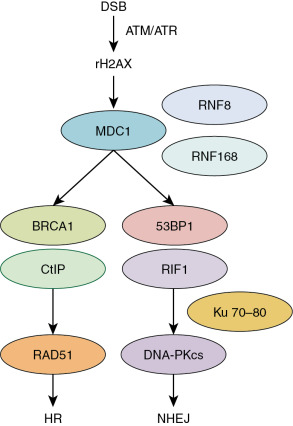
Taken together, the overall DNA damage caused by proton is different than that of photon beams, at least to a certain extent. However, the repair mechanisms of the DSBs induced by proton beams are still unclear. Future studies specifically investigating the DNA repair pathways of proton beams will translate the findings into biology-based rationales of treatment selection between proton- and photon-based radiation and the combination of therapies that targeted specific signal pathways.
Cell death
One of the severe consequences of the failure of DNA damage repair induced by radiation is cell death. The mechanisms of photon radiation–induced cell death are intensively studied. Photon radiation is known to kill cancer cells via apoptosis, necrosis, autophagy, mitotic catastrophe, and senescence. However, the mechanisms by which proton radiation induces cell death are unclear.
Cell mitotic catastrophe
Extensive evidence demonstrated that mitotic catastrophe is the major mechanism of cell death in solid tumors in response to photon radiotherapy. , However, the role of cell mitotic catastrophe in response to proton beam radiation is unclear. We uncovered that mitotic catastrophe was the dominant mechanism of cell death in both human papillomavirus (HPV)–related and HPV-unrelated human head and neck squamous carcinoma cells after proton beam radiation at 4, 24, 48, and 72 hours after radiation ( Fig. 1.2 ). Moreover, the results demonstrated that compared with photon beams, a 4-Gy dose of proton radiation led to a higher level of mitotic catastrophe in these cells. The more pronounced cell mitotic catastrophe induced by proton beams versus photon beams suggests that combining therapy targeting the DNA damage repair pathway may promote cell death differently after proton radiation compared with photon radiation.
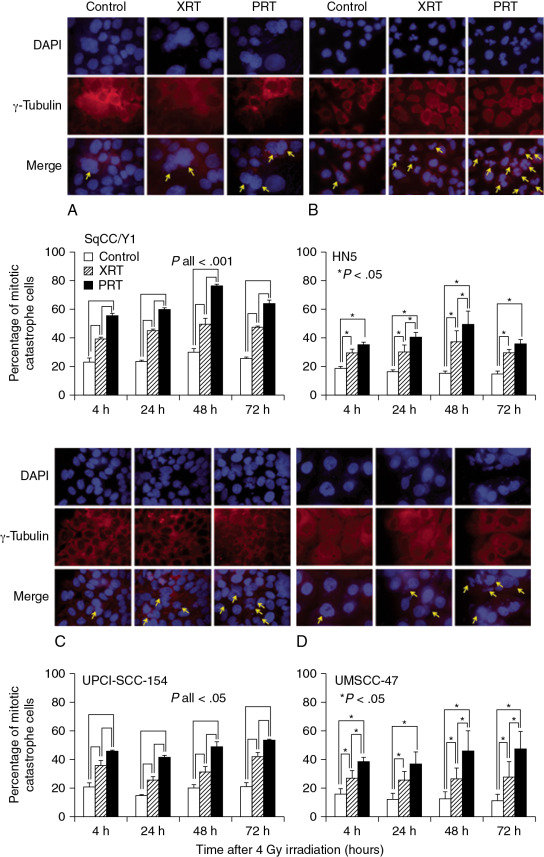
Cell senescence
With emerging evidence, cellular senescence is increasingly being recognized as one of the most important mechanisms in photon radiation–induced tumor suppression. , , Similar to that observed in photon radiation, we found that senescence was also a major type of cell death induced by a 4-Gy dose of proton beam radiation in HPV-related and HPV-unrelated human head and neck squamous carcinoma cells at 4 and 6 days after exposure ( Fig. 1.3 ). More importantly, compared with photon beams, proton beams led to a higher proportion of cells undergoing senescence in these cell lines. Based on the above facts, the role of combination treatment that interferes with cell senescence pathways may influence cell responses to proton beams versus photon beams differently and warrants further investigation.
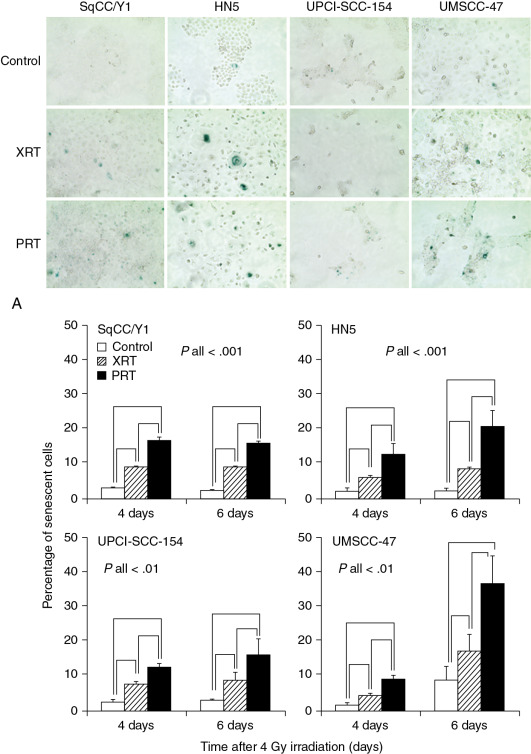
Cell apoptosis
Apoptosis plays a modest role in the response of many solid tumors to photon irradiation. To date, little is known about cell apoptosis after they are exposed to proton radiation. The study result from one group indicated that compared with photon beams, proton beams led to a greater level of cell apoptosis at 48 hours after radiation in H460 and A549. , Similarly, a study (16 in DNA damage literature) using patient-derived glioma stem cells to compare proton beam with photon beam irradiation indicated that proton beams induce more cell apoptosis and lead to more cell apoptosis–related caspase-3 activation and poly(adenosine diphosphate [ADP]-ribose) polymerase (PARP) cleavage. Other than the higher incidence of cell apoptosis after proton beam versus photon beam radiation, studies from other groups also revealed the time point differences of the cell apoptosis occurrence between photon versus proton beam radiation. One group from Italy exposed the prostate adenocarcinoma cell line PC3 to photon and proton beams. They found that the peak of PC3 cells undergoing apoptosis was reached at 8 hours after proton irradiation compared with 48 hours for photon irradiation. Differently, a study from Germany on HeLa cells indicated that during the maximum observation time of 48 hours, the proportion of apoptotic cells induced by proton beams increased with time. Other than the above direct evidence of cell apoptosis induced by proton beams, indirect evidence of cell apoptosis–related signal pathway changes were also generated. One study demonstrated significantly more upregulation of proapoptotic gene, Bax , and downregulation of antiapoptotic gene, Bcl-2 , at 12 hours after lung cancer A549 cells were exposed to proton beams compared with those cells exposed to photon beams. However, our study in HPV-related and HPV-unrelated human head and neck squamous carcinoma cells showed a different result ( Fig. 1.4A ). Both photon and proton beams only induced limited cell apoptosis, and no difference was observed in the proportion of proton beam–induced cell apoptosis versus that induced by photon beams. Because both proton beams and photon beams can cause DNA damage and DNA damage is a major pathway by which radiation causes apoptosis, strategies to target apoptosis pathway to enhance proton beam or photon beam–induced tumor cell apoptosis may be another effective strategy for enhancing the antitumor activity of radiation.
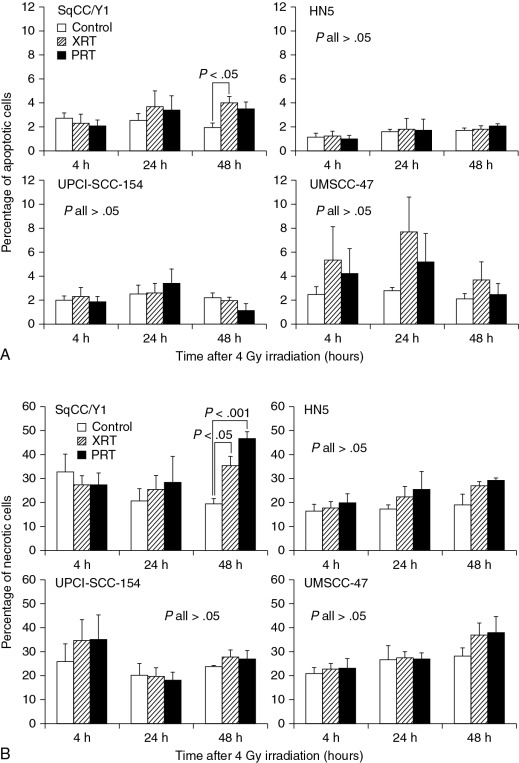
Cell necrosis
Necrosis typically occurs after a large dose of photon radiation, , but it has also been observed in cancer cell lines and patient tumor tissue–derived cancer cells after a single 4-Gy or 6-Gy dose of photon irradiation. Comparing the proportion of cells undergoing necrosis in four HPV-related and HPV-unrelated human head and neck squamous carcinoma cell lines after a single 4-Gy dose of proton or photon beam radiation, we found that proton and photon beams only led to significantly increased necrosis in one HPV-unrelated cell line 48 hours after radiation, and no differences were found between proton versus photon beams ( Fig. 1.4B ). Mechanistic studies of tumor necrosis have identified several molecular targets that mediate necrosis after treatment. Interfering with those molecular targets may be another new approach to promote both proton and photon beam–induced necrotic cell death and may be a potential to enhance radiosensitivity. ,
In summary, mitotic catastrophe and senescence are the major types of cell death induced by both photon and proton beams, and proton beams kill more cells by either mechanism than photon beams. Individual cancer patients with different gene mutation statuses may derive different levels of benefit from targeted therapy that interferes with different cell death–related pathways according to whether the radiotherapy is photon or proton based. Further mechanistic and in vivo studies may open a new avenue of improving tumor control with proton or photon radiation and lead to novel, individually optimized combination treatment plans consisting of molecular-targeted therapy combined with proton or photon beams for cancer patients with tumors of different biological features.
Relative biological effectiveness
Proton therapy has shown promise to protect normal tissues in the treatment of malignant diseases such as pediatric cancers, central nervous system and skull base tumors, ocular melanoma, , and head and neck cancers that are near critical structures and are difficult to treat with surgery or conventional photon radiation. , However, because of variations in the RBE of protons in different types of cells or tissues, , whether the dosimetric advantages of proton beams can be translated into demonstrable clinical benefits of normal tissue protection and tumor control for these cancers remains unclear.
Currently, clinical use of proton beams is based largely on the experiences that are derived from photon beam radiation. However, the difference in the energy deposition patterns of photon beams and proton beams means that equal doses of proton or photon beam radiation do not produce equal biological effects; one type of radiation may be more effective at killing cells than the other one. The RBE of proton beams is defined as the ratio of the doses required for photon versus proton beams radiation to produce the same level of biological effectiveness, such as cell killing or DNA damage. , The RBE of proton beams has been recognized as variable values. The RBE is determined by a number of physical and biological factors, such as proton beam energy, depth, radiation dose, radiation fraction size, radiation fraction number, cell or tissue types, and the end points. , The advantages of proton versus photon beams only can be presented in the case of accurately assured higher/equal target volume dose and lowered surrounding normal tissue dose in proton radiation. Therefore an accurate proton beam RBE is required in proton beam radiation.
In current clinical practice, the RBE of proton therapy has been assumed to be 1.1 regardless of tumor type, beam energy, and treatment planning differences. This RBE value was mainly derived from preclinical experiments with normal cells or early-reacting normal tissues rather than cancer cells or tumor xenografts. Moreover, these experiments also demonstrated a big range of RBE at the middle of SOBP (ranging from 0.9 to 2.1 for in vitro experiments and from 0.7 to 1.6 for in vivo experiments). Thus, use of this constant RBE without considering differences in tumor biology or the effects of fractionation increases the extent of clinical dose uncertainties for tumor and normal tissues associated with proton therapy, the nature and extent of which are largely unknown but are crucial to the safe and effective use of proton therapy.
More importantly, emerging evidence has established the increased RBE values in regions of high LET at the distal falloff of most proton beams, which are normally located within the target volume. Thus, special attention should bring in treatment planning of proton beam radiation to avoid locating organs at risk to the distal portion of SOBP, which might induce normal tissue complications at the distal field edges. Furthermore, because in vivo study also found a trend that late-responding tissues may have higher RBE values compared with early-responding tissues, more attention should be placed on the observation of late normal tissue response in those patients who accepted proton beam radiation in the clinical setting.
In summary, because of the uncertainties of the RBE in proton beam radiation, more studies on the clinically relevant dose range in the response of different normal tissues or tumor types in animals are needed.
Immune response
Accumulated evidence has demonstrated that photon beam radiation not only can control tumor by local tumor irradiation but can also influence tumor growth by the effects of photon radiation on the activation and suppression of the immune system ( Fig. 1.5 ). To avoid the immunosuppression effect and to enhance the immunoactivation effect of photon radiation, the impact of photon radiation on the immune system is under extensive study currently to investigate the possibility of radiation and immune therapy combination to improve cancer treatment outcomes.
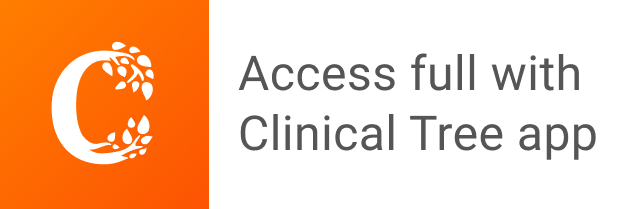