Chapter 9
Motion, Pulsation, and Other Artifacts
Kimball L. Christianson, Phil B. Hoang, Steve Huang, Mark L. Lessne, Allen W. Song, and Elmar M. Merkle
CASE 1
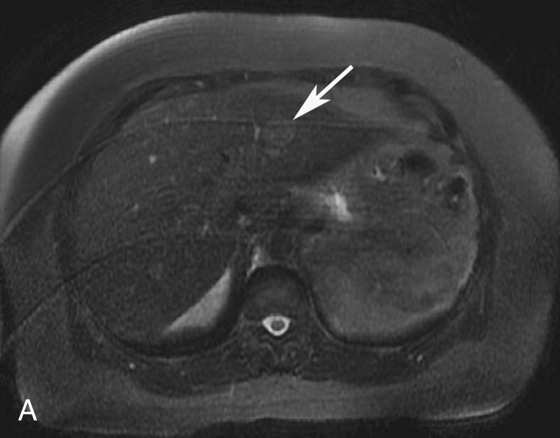
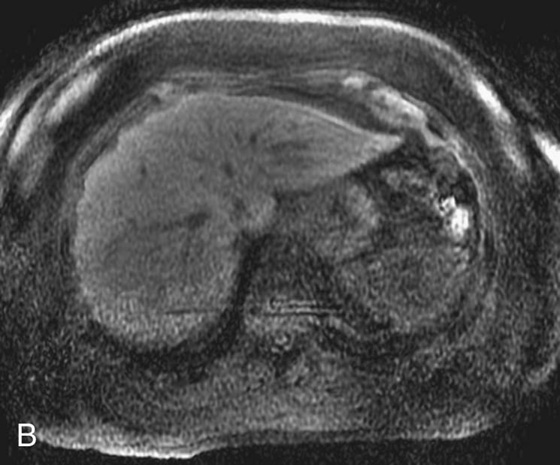
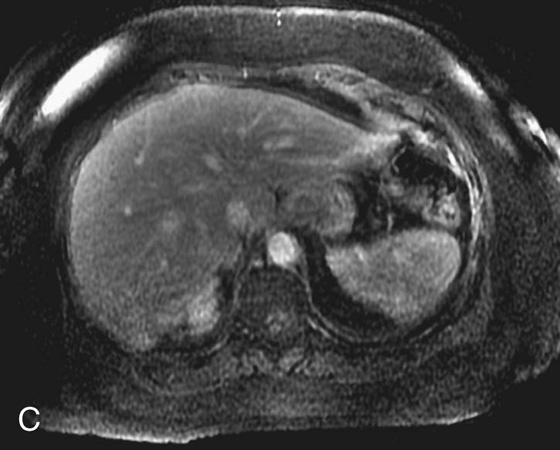
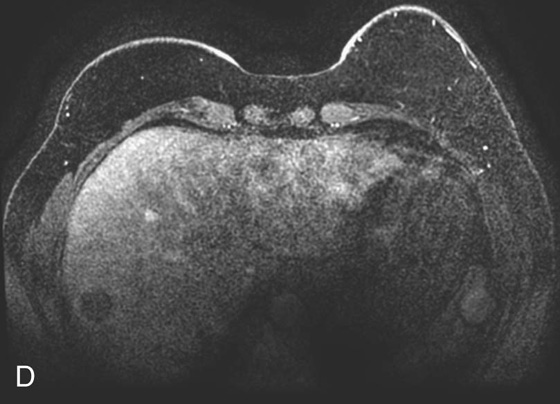
1. What is the differential diagnosis for the bright lesion in the left hepatic lobe in Figure 1A?
2. What is the finding in Figure 1D (different patient)? What other finding in the images suggests the lesion in the right hepatic lobe is artifactual?
3. What is this artifact called and what causes it?
4. Why is the artifact oriented vertically in Figures 1A-C and horizontally in Figure 1D?
ANSWERS
CASE 1
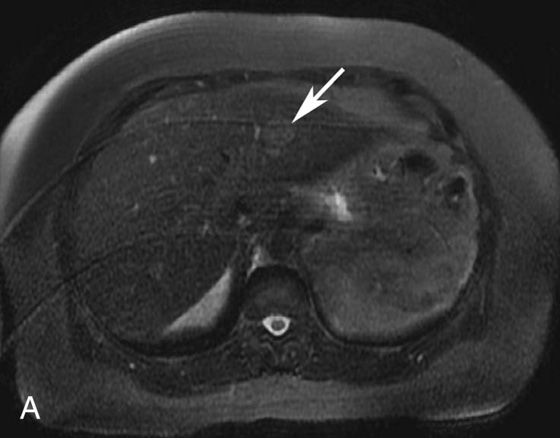
FIGURE 1A. Axial fat-suppressed T2-weighted image of the upper abdomen demonstrates a high signal intensity, round lesion in the left hepatic lobe (white arrow). This lesion is not seen on the pre- and postcontrast fat-suppressed three-dimensional (3D) gradient-recalled echo (GRE) axial T1-weighted (T1W) sequences.
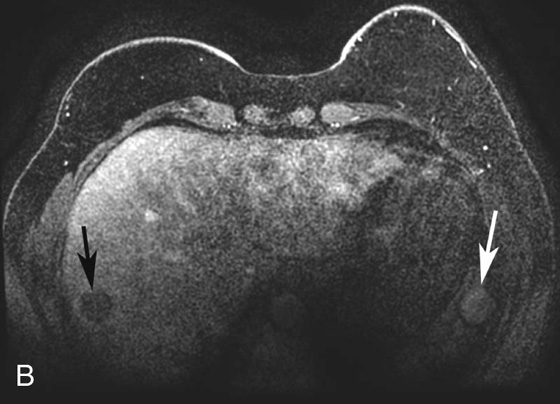
FIGURE 1B. Axial fat-suppressed T1W 3D-GRE sequence from a dedicated breast magnetic resonance (MR) image (different patient) demonstrates a round, low-signal lesion in the right hepatic lobe (black arrow) and a round high-signal lesion in the region of the spleen/abdominal wall (white arrow).
1. The differential diagnosis of a subtle, T2 bright lesion in the liver includes hemangioma, metastatic disease, and primary liver tumor. However, given its location, oriented vertically just above the aorta, a pseudo-lesion as a result of pulsation artifact from the aorta is also in the differential.
2. Round low-signal lesion in the right hepatic lobe. There is a subtle high-signal lesion located equidistant to, but left of the aorta along the same horizontal plane as the low-signal lesion in the right hepatic lobe.
3. This artifact is referred to as a “ghosting artifact.” Ghosting artifacts appear in abnormal locations as replicas of the moving structure from which they result. They are observed only in the phase-encoding direction and can be the result of any periodic motion such as respiration, arterial pulsation, or cerebrospinal fluid pulsation.
4. Figures 1A through 1C are from an abdominal MR image, and the phase-encoding direction is oriented vertically; therefore, the artifact is also seen along this axis. Figure 1D is from a breast MR image in which the phase-encoding direction is oriented horizontally from right to left.
Part 1: Motion and Pulsation Artifact
Motion is a very common cause of artifacts in magnetic resonance imaging (MRI). Motion artifacts are the result of movement during the data acquisition period. More specifically, when motion is present, tissues excited at a specific location during the radiofrequency (RF) pulse are erroneously mapped to a different location (or often multiple different locations in cases of motion artifacts) during detection. Motion is often divided into two categories: gross body movement and physiologic motion, such as cardiac and respiratory cycles, or blood or cerebrospinal fluid (CSF) flow. In most conventional imaging methods, motion artifact is predominantly manifested in the phase-encoding direction.
The extensive use of spatial gradients complicates and amplifies motion artifacts, as stronger gradients induce larger phase shifts from motion (see Understanding the Need for Gradients In Image Formation and Its Implication in Motion Artifacts). While some gradient combinations can compensate for motion (e.g., the flow-compensated gradients; see below for more details), many of the imaging gradient pulses cannot. As such, the inconsistent nature of motion can induce different phase shifts during the image readout period. When viewed from the phase-encoding direction in the final data space, these inconsistencies introduce local deviations that result in ghosting artifacts in the image space as the result of Fourier transformation. For example, a pulsatile effect in one of the data lines (along the frequency-encoding direction) would be viewed as a spike along the phase-encoding direction, which would then result in a streaking line artifact along the phase-encoding direction. More severely, several inconsistent data lines would result in more extensive ghosting artifacts.
Understanding the Need for Gradients in Image Formation and Its Implication in Motion Artifacts Why are gradients necessary? If an RF pulse were broadcast without gradients, then every proton along the main magnetic field would be excited. The receiver coil would receive signals from the protons all resonating at the same frequency and with the same phase, making spatial localization and, therefore, a coherent magnetic resonance (MR) image impossible.9 The three magnetic field gradients required to form an image are the slice selection, frequency-encoding, and phase-encoding gradients. The primary purpose of these gradients is to assign spatial localization to the resultant MR image. For an axial image, the slice selection gradient assigns location in the z direction, the frequency-encoding gradient, or readout gradient, assigns location in the x direction, and the phase-encoding gradient assigns location in the y direction. |
The purpose of a slice selection gradient is to expose protons along the z-axis to a different magnetic field, inducing the protons to precess at different frequencies while administering an RF pulse with a very narrow range of frequencies, resulting in excitation of only a thin slice of tissue along the z-axis. Only those protons along the z-axis gradient that have frequencies corresponding to the RF pulse are excited.9 The range of frequencies transmitted by the RF pulse is referred to as the transmitter bandwidth. The slice thickness can be made thicker or thinner by adjusting the gradient strength or the transmitter bandwidth. Increasing the gradient strength or lowering the transmitter bandwidth results in a thinner slice. Decreasing the gradient strength or increasing the transmitter bandwidth results in a thicker slice. |
The frequency-encoding gradient is critically important for spatial localization along the x-axis. Unlike the slice selection gradient, which is applied at the same time as the RF excitation pulse, the frequency-encoding gradient is applied concurrent with echo sampling. The frequency-encoding gradient induces protons along the x-axis at different locations to precess at different frequencies. For example, the protons on the right side of the body will precess a little faster than protons on the left side of the body. With use of the Fourier transform, these differences in frequency can be translated into differences in signal at each spatial location to create the MR image. |
The frequency-encoding gradient is also important in the generation of an echo. Over time, proton spins in the presence of a spatial gradient would accumulate phase shifts. The generation of MR signal is dependent on the protons being in phase at just the right time during echo sampling. Gradients’ dephasing properties are manipulated in a controlled fashion such that phase coherence can be achieved and an echo generated. This is accomplished by applying a gradient with two lobes that have opposite polarity. The first lobe of the gradient is the dephasing lobe. A second gradient is then applied with opposite polarity and typically twice the duration, called a rephasing lobe. At the midpoint of this rephasing lobe, the sampled protons are most in phase and an echo is generated, referred to as a gradient-recalled echo. If a 180° refocusing pulse is applied prior to the rephasing lobe and after 90° excitation pulse, the generated echo is referred to as a spin echo. Pulse sequences are usually timed so that the gradient-recalled echo and the spin echo occur at the same time. In the case of a spin echo acquisition, the rephasing lobe should be applied with the same polarity (rather than the opposite polarity) as the dephasing lobe because the 180° refocusing pulse results in a complete reversal of phase. The direction of the frequency-encoding gradient is almost always applied along the axis with the widest dimension (e.g., right to left in the abdomen and anterior to posterior in the head).10 |
Finally, a phase-encoding gradient is used for spatial localization along the y-axis. In contrast to the frequency-encoding gradient, the phase-encoding gradient is applied right before the data acquisition (but also after the slice excitation). As such, the protons would have already experienced the gradient and accumulated certain controlled phase shifts. Since the phase-encoding gradient is turned off before the data readout, these phase shifts would remain fixed to ensure the same amount of phase encoding. To complete the coverage for a two-dimensional (2D) image, for example, different phases are assigned with different phase-encoding gradient amplitudes prior to the data acquisition window. A subsequent Fourier transformation can then be used to spatially resolve the image along the phase-encoding direction. It is worth noting that a rephasing gradient (such as that used in slice selection) or a dephasing gradient (such as that used in frequency encoding) is not usually needed for phase encoding. Spatial localization is encoded from differences in phase rather than frequency by applying the phase-encoding gradient after the initial excitation pulse and before echo sampling.9 |
Spatial encoding along the frequency-encoding direction can be performed in its entirety with a single RF excitation pulse. Along the phase-encoding axis, spatial localization usually (save for single-shot imaging techniques, such as echo-planar imaging) requires the application of numerous phase-encoding gradients, each with a different strength, with each new RF excitation. The number of phase-encoding steps required determines the extent of the MR image along the y-axis and, along with the time to repetition (TR), is an important contributor to image acquisition time. Strong phase-encoding gradients create larger differences in phase and allow better discrimination of objects that are close together (better spatial resolution) in the resultant MR image. The down side of a stronger (i.e., steeper) gradient is that protons on one end of the gradient are more out of phase with protons at the other end of the gradient, thereby reducing signal and contrast. Weaker gradients result in better signal and contrast. This principle has important implications in the way k-space is filled. By convention, the center of k-space is the high-contrast region, and the periphery contributes to the fine detail and spatial resolution of the image. It makes sense, then, that the center of k-space is filled first with echoes resulting from the weaker phase-encoding gradients, and the gradient strength increases gradually as k-space is filled from central to peripheral.9 |
In short, motion artifacts are predominantly manifested along the phase-encoding direction. More specifically, random motion results in smearing or blurring in the phase-encoding direction, and periodic motion (cardiac motion and blood vessels) results in ghosting artifacts. Ghosting artifacts appear as replicas of the moving structure at specific intervals along the phase-encoding axis. The motion artifact patterns are dependent on how repeatable the phase shifts were along the phase-encoding direction in k-space. For example, if only one line deviates, then the artifact will be a solid line across the MR image. If it repeats every other line, then the artifact will be a displacement over half the field of view. If all the lines deviate the same way (e.g., after excessive averaging), then there will appear to be no artifact.11
Why does flowing blood cause motion artifacts? After application of the dephasing lobe of the slice selection and frequency-encoding gradients, blood moves to a different location and experiences a rephasing gradient of a different strength. The phase shift induced by the dephasing lobe cannot be reversed by a gradient of a different strength, and the phase difference persists as ghost artifacts.10
Numerous methods can be used to reduce motion artifacts. Increasing the sampling bandwidth (recall the relationship bandwith = 1/time for echo) is a simple method to reduce motion artifact at the expense of the signal-to-noise ratio. While it is true that increasing the gradient strength or the time in which the gradient is applied will increase susceptibility to motion artifact, increasing the strength of the frequency encoding gradient is an exception. The sampling bandwidth increases with increasing (steeper) gradient strength. A steeper gradient means that rephasing of the protons happens more quickly and an echo forms faster, thereby decreasing sampling time.10
Another method to reduce motion artifact is called gradient moment nulling. When motion occurs during either the dephasing or rephasing lobe of the gradient, there is incomplete phase cancellation, which leads to a net accumulation of phase referred to as the gradient moment. This phase accumulation can be the result of protons moving with constant-velocity motion (first-order motion), acceleration (second-order motion), or pulsatile or jerk motion (third-order motion). In its simplest form, an applied gradient without motion correction is a unipolar gradient. Application of additional gradient pulses, such as in the form of a bipolar gradient, can rephase the phase shift from both stationary and moving tissues and significantly reduces first-order or constant-velocity motion. Application of more complex gradient pulses can also reduce second- and third-order motion; however, gradient moment nulling works best for first-order motion. Gradient moment nulling requires a longer time to echo (TE); with first-order nulling the increase in TE is negligible, but with second- or third-order nulling, the longer TE can be problematic.11
Switching the direction of the frequency- and phase-encoding gradients is a simple way to manipulate motion artifact. Motion artifact is not eliminated with this method, but rather is displaced along another axis. This method can be very helpful in trying to distinguish whether a finding represents true pathology or is due to motion artifact.
Presaturation pulses are another often-utilized method to reduce the effects of motion artifact. This technique is a preferred method if the signal to be nulled is not necessary for image interpretation. Presaturation pulses can be used to null fat if its signal is contributing to the motion artifact. It can also be used to saturate the protons in flowing blood before it enters the volume of tissue being imaged, and is a frequently used technique in time-of-flight (TOF) imaging (see Chapter 11).10
Averaging is a motion reduction technique often used to eliminate ghosting artifacts caused by respiratory motion. This method takes advantage of the fact that, on average, normal tissue stays in a relatively constant location with each respiratory cycle while the location of the ghosting artifacts is much more variable with each breath. Averaging is typically accomplished by acquiring more than one signal with each phase-encoding step. The average signal of tissue is much more coherent and contributes more to the overall appearance of the image when compared to the signal produced from the ghosting artifacts.10
Respiratory triggering is a method used to decrease respiratory motion artifact. This is accomplished either with a bellows on the upper abdomen that tracks the motion of the respiratory cycle or with a “Navigator” technique, which produces a signal that indicates the position of the diaphragm. Typically the signals are acquired during end expiration with each cycle. Image acquisition takes longer with this technique since signal acquisition is restricted to end expiration. Cardiac gating synchronizes image acquisition with the electrocardiogram, which is very useful in eliminating cardiac motion artifact.
Perhaps the most effective method to reduce respiratory motion is respiratory suspension. Single breath-hold techniques with 2D multislice or three-dimensional (3D) acquisitions can be performed on patients capable of holding their breath. For patients who have difficulty holding their breath, ultrafast imaging techniques can be helpful in producing diagnostic images.10
CASE 2
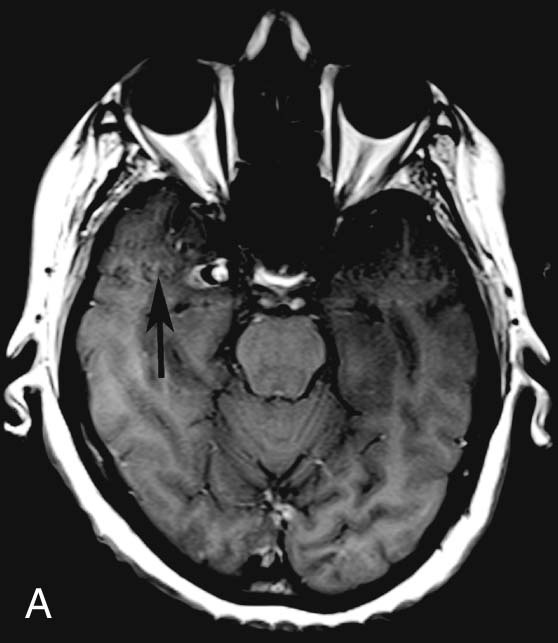
FIGURE 2A. Axial T1W image of the head demonstrates a focal lesion along the medial aspect of the right temporal lobe with central high T1 signal and peripheral low T1 signal. Ghosting artifact is seen on both sides of the lesion extending in the phase-encoding direction (black arrow).
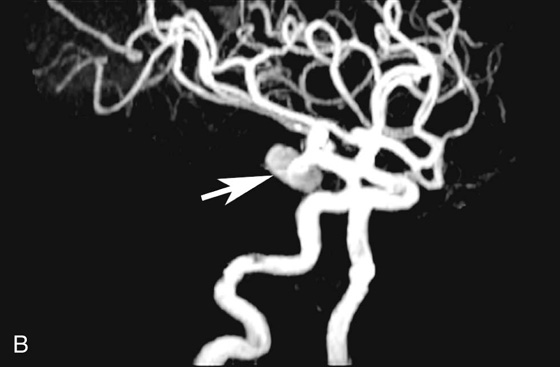
FIGURE 2B. Maximum intensity projection (MIP) image of the circle of Willis from a time-of-flight (TOF) magnetic resonance angiogram (MRA). There is a saccular outpouching seen adjacent to the right supraclinoid internal carotid artery (ICA) (white arrow).
Diagnosis:
Right supraclinoid internal carotid artery (ICA) aneurysm.
Discussion
It is extremely important to consider the diagnosis of an aneurysm when developing a differential for an extra-axial parasellar mass. Thrombus within a cerebral aneurysm is composed of blood products of different ages, resulting in the classic appearance of a central flow void with circumferential rings of varying signal intensity extending peripherally. The diagnosis is clinched with recognition of pulsation artifact in the phase-encoding direction, which is due to pulsatile blood flow within the aneurysm. However, lack of artifact does not exclude an aneurysm, since completely thrombosed aneurysms will not produce pulsation artifact. The protons within the moving blood experience a rephasing gradient of a different strength and accumulate phase, which results in the ghosting artifact seen above.
CASES 3 AND 4: COMPANION CASES
Case 3
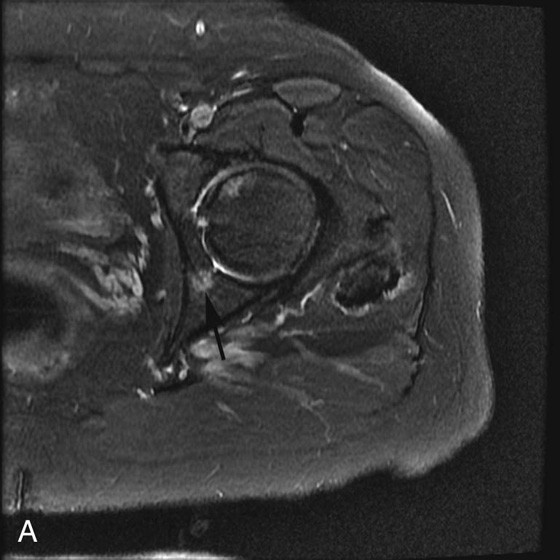
FIGURE 3A. Axial fat-suppressed T2-weighted (T2W) image of the left hip in a patient with breast cancer. Round, high-signal focus is identified in the left posterior acetabulum (black arrow).
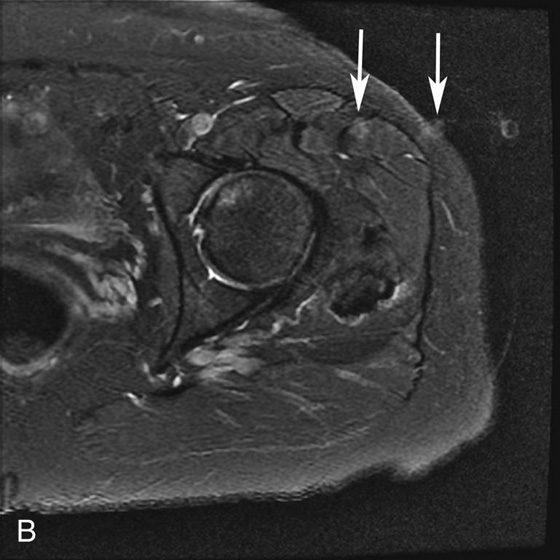
FIGURE 3B. Axial fat-suppressed T2W image in the same patient. The frequency- and phase-encoding directions were swapped; the high-signal acetabular focus seen in Figure 3A is no longer identified. Note the vascular pulsation artifact arising from the left femoral artery (white arrows).
Diagnosis:
Pseudo-lesion from pulsation artifact.
Case 4
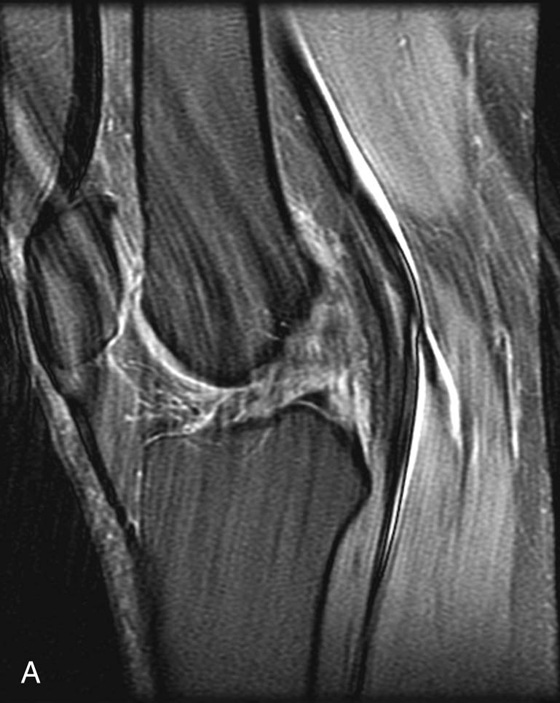
FIGURE 4A. Sagittal fat-suppressed proton density (PD)–weighted image demonstrating extensive ghosting artifact resulting from pulsation of the popliteal artery.
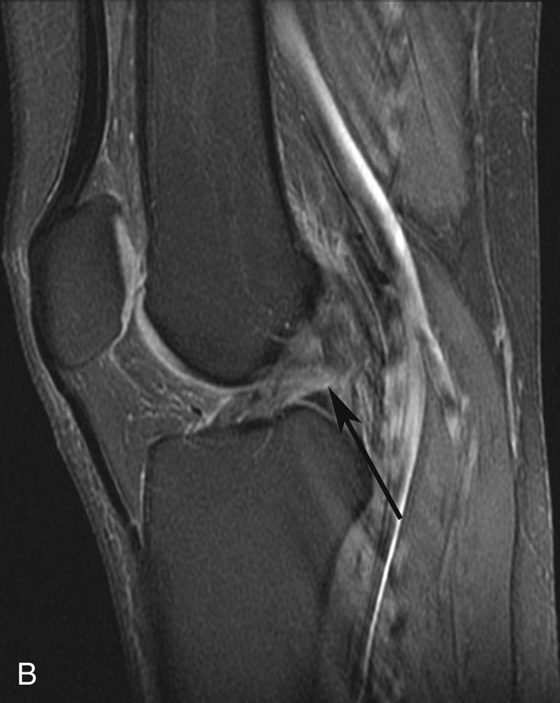
FIGURE 4B. Sagittal fat-suppressed PD-weighted image in the same patient. The frequency- and phase-encoding directions were swapped, resulting in significantly decreased artifact. The anterior cruciate ligament (ACL) tear is now much more easily seen (black arrow).
Diagnosis:
Anterior cruciate ligament (ACL) tear obscured by pulsation artifact from the popliteal artery.
Discussion
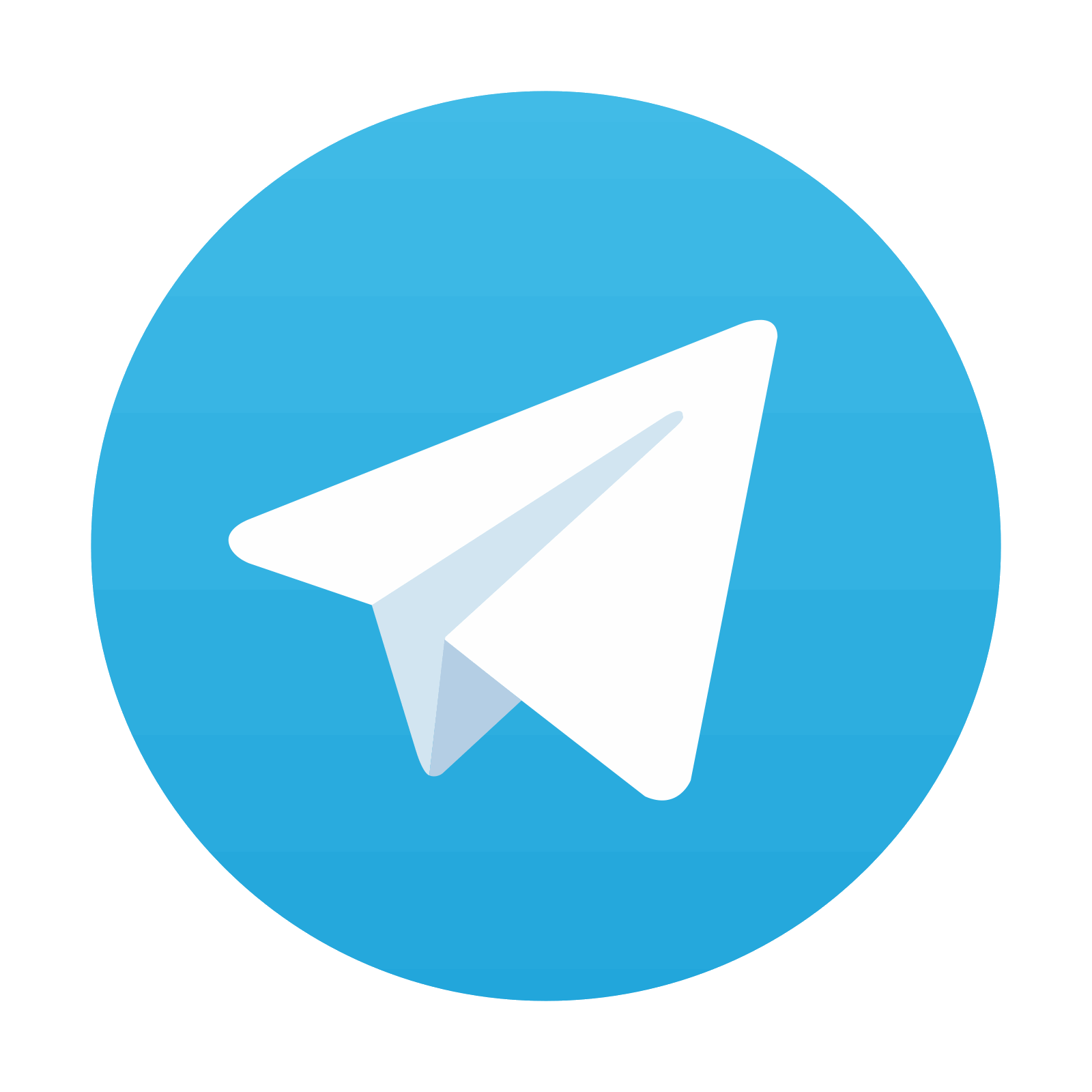
Stay updated, free articles. Join our Telegram channel
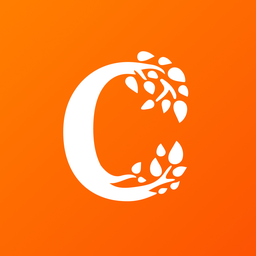
Full access? Get Clinical Tree
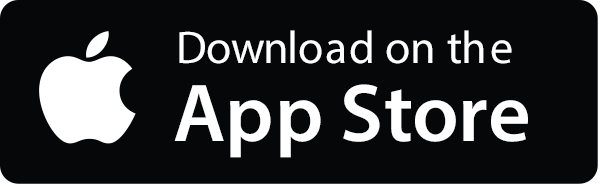
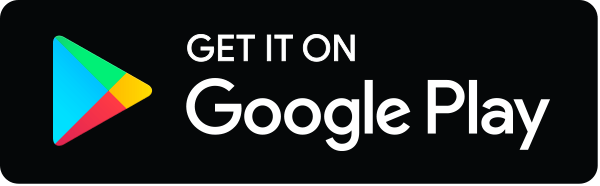