© Springer International Publishing Switzerland 2017
Philip J. Tofilon and Kevin Camphausen (eds.)Increasing the Therapeutic Ratio of RadiotherapyCancer Drug Discovery and Development10.1007/978-3-319-40854-5_22. Receptor Tyrosine Kinases as Targets for Enhancing Tumor Radiosensitivity
(1)
Department of Therapeutic Radiology, Yale University School of Medicine, 208040, New Haven, CT, USA
Abstract
The advent of the modern era of molecularly targeted therapies in oncology has generated considerable excitement in the field of oncology. While there have been successes with molecularly targeted agents as monotherapies, most solid tumors display only a transient and modest response to single-targeted agents. As such, there has been significant effort in combining molecularly targeted agents with radiotherapy. Receptor tyrosine kinases (RTKs) play central roles in oncogenesis, stress sensitivity, tumor maintenance/progression, and clinical prognosis. Secondary to these roles, receptor tyrosine kinases are attractive targets for cancer therapy and specifically in combination with radiation therapy to enhance tumor radiosensitivity. Significant preclinical and clinical investigations have been performed to understand their roles in regulating the cellular response to radiation. A number of RTKs with relevance to radiation oncology have been identified including EGFR, VEGFR, IGF-1R, c-MET, and HER2. This chapter will highlight the preclinical and clinical findings associated with the combination of radiotherapy and inhibitors of the aforementioned receptors.
Keywords
RadiosensitizationReceptor tyrosine kinaseEGFRVEGFc-MetFGFRHer2Epidermal growth factor receptorRTKIntroduction
Clinicians have long combined radiation therapy with systemically delivered agents to enhance the local effects of RT, improve tumor control, and enhance patient survival. This combined modality approach couples standard fractionated radiation treatment regimens with cytotoxic chemotherapies such as 5FU, mitomycin , cisplatin , taxol , and gemcitabine . While these combinations have shown success for specific disease sites in the clinic, substantial limitations exist. Chief among these are the dose limitations and toxicity imposed by normal tissue responses to the nonspecific nature of cytotoxic chemotherapy.
The advent of the modern era of targeted therapies has been met with great excitement in the oncology community. By attacking aberrantly activated pathways only present in tumor cells, targeted therapies have the potential benefit of being able to minimize normal tissue toxicity while maximizing tumor effect. While there have been successes with the use of targeted agents as monotherapies (e.g., imatinib in the BCR-Abl-driven chronic myeloid leukemia) [1], most common solid tumors have shown only a modest and transient response to single-targeted agents [2]. As such, tremendous effort has been expended in studying the combinations of these molecularly targeted agents with standard chemotherapies and/or radiation.
One target-rich area of tumor biology that has received considerable interest is membrane receptor (or specifically receptor tyrosine kinase) signaling. These kinases have been shown to play an important role in oncogenesis, stress sensitivity, tumor maintenance/progression, and clinical prognosis [3]. Secondary to these roles, receptor tyrosine kinases (RTKs) are attractive targets for cancer therapy and specifically in combination with radiation therapy to tumor radiosensitivity. As such, considerable preclinical and clinical investigations have been performed to understand their roles in regulating the cellular response to radiation. A number of RTKs with relevance to radiation oncology have been identified including EGFR, VEGFR, IGF-1R, c-MET, and HER2 [2, 4, 5]. This chapter will highlight the substantial preclinical and clinical findings associated with the combination of radiotherapy and inhibitors of the aforementioned receptors.
EGFR
The erbB family of receptors has been the subject of extensive laboratory and clinical investigations. The erbB family consists of four distinct receptors: EGFR (erbB1), HER-2/NEU (erbB2), erbB3, and erbB4 [6]. EGFR or epidermal growth factor receptor is the most well studied of the family with regard to its role in modulating a tumor’s response to radiation.
The EGFR is a 170-kDa transmembrane RTK that plays an important role in carcinogenesis , tumor progression , and response to therapy [6]. Structurally, EGFR is comprised of four extracellular domains, a hydrophobic transmembrane domain, a juxtamembrane sub-domain, an intracellular tyrosine kinase domain , and c-terminal phosphorylation sites [6]. The natural ligands of the EGFR include epidermal growth factor (EGF) , transforming growth factor alpha (TGF-α) , epiregulin , betacellulin , amphiregulin , and heparin-binding EGF-like growth factor (HB-EGF) [7]. The EGFR is present in a monomeric state , but ligand binding drives a conformational change of the extracellular domain that causes receptor homo- and heterodimerization with other ErbB family receptors [8]. This dimerization activates intracellular tyrosine kinase domain auto- and transphosphorylation and initiates downstream signal transduction [8]. The EGFR and other RTKs are also activated by ionizing radiation [9]. The mechanisms that underlie this phenomenon include (1) receptor clustering and dimerization [10, 11], (2) radiation-induced release of autocrine ligands [12], and (3) phosphatase inactivation [13].
Signal transduction downstream of the EGFR occurs through a number of critical pathways including RAS/RAF/MAPK, PI3K/AKT/mTOR, Jak/STAT, Src, and PLC-DAG/PKC [14–18]. While the goal of this chapter is not to describe in detail each of these pathways, it is important to highlight their respective roles in the radiation response and tumor biology in general. The PI3K/AKT/mTOR pathway has been shown to be directly involved in regulating cell survival after radiation both in vitro and in vivo [19–21]. Various investigations have demonstrated different mechanisms by which this pathway governs radiosensitivity: through regulation of metabolic demands through activation of the mechanistic target of rapamycin (mTOR) kinase , control of proliferative signaling via MAPK cascade stimulation, and activation of cell survival signaling through AKT [22]. Other work has also documented the roles of Jak/STAT and PKC pathways in influencing tumor cell radiosensitivity [23, 24]. Ultimately activation of these pathways modifies cellular responses and repair programs induced by DNA damage, and regulation of these critical oncogenic pathways by EGFR underscores its potential as a target for enhancing tumor radiosensitivity and improving patient outcomes.
EGFR has a well-documented role in cancer [6] that was initially implicated by increased expression levels in a wide range of cancers including ovarian, brain, breast, colorectal, non-small cell lung cancer (NSCLC) , and head and neck squamous cell carcinomas (HNSCC) [25, 26]. Based upon this appreciation, several classes of EGFR inhibitors have been developed. These inhibitors belong broadly to two classes: monoclonal antibodies (mAb) that target the extracellular ligand-binding domain and small molecule inhibitors that target the intracellular kinase domain [2, 27]. mAbs to the EGFR recognize, inactivate, and remove the receptor from the cell surface, and several mAbs have been advanced to the clinic including cetuximab , panitumumab , and matuzumab [2, 27]. Cetuximab is FDA approved for the treatment of HNSCC in combination with radiation [2, 5, 27]. Small-molecule tyrosine kinase inhibitors (TKIs) , which bind to the intracellular ATP-binding domain of the EGFR, prevent receptor phosphorylation and subsequent signal transduction [2, 27]. A number of these TKIs have been developed and tested in the laboratory and clinic. Gefitinib and erlotinib are two EGFR -specific TKIs developed as single agents for advanced NSCLC and that have demonstrated efficacy in clinical trials [28, 29].
The observation that prolonged exposure of head and neck cancer cells to EGF enhanced the effects of radiation by clonogenic survival began to spark interest in studying the effects of EGFR modulation and radiation [30, 31]. While these initial in vitro results seem counterintuitive, it is likely that prolonged EGF exposure resulted in EGFR internalization and degradation causing a decrease in EGFR signaling. Another early study by Balaban et al. showed that targeting of EGFR via the anti-EGFR antibody LA22 resulted in an increase in radiation-induced apoptosis [32]. Additionally, several other groups demonstrated in preclinical models (in vitro and in vivo) that EGFR expression inversely correlated with radiation sensitivity [33–35]. This correlation was also observed in clinical samples , and in fact poor survival of HNSCC patients with high EGFR tumors was shown to be secondary to poorer local regional tumor control and not distant metastasis [36]. The in vitro observation that radiation activates EGFR receptor phosphorylation [9, 37] and several downstream signaling cascades such as Ras/MAPK and PI3K/AKT/mTOR [17, 38] provided a mechanistic rationale for targeting EGFR function concurrent with RT, and genetic models of EGFR blockade indeed provided evidence that radiosensitization could be achieved through EGFR inhibition [10, 39].
These initial preclinical results, as well as parallel work examining EGFR targeting as a monotherapy , led to the development of the mAb, C225 (now known as cetuximab ). C225 was shown to enhance radiation effects in vitro in HNSCC cell lines despite also causing a G1 cell cycle arrest, a finding that supported the potential for clinical translation [40]. Preclinical and clinical research has also been performed on additional mAbs such as mAb806, which recognizes an activation-specific conformation of the receptor. This antibody has been shown to bind a cryptic EGFR epitope that is exposed in the presence of oncogenic mutations such as EGFRvIII or is coincident with overexpression and activation of wild-type EGFR [41]. The specificity of blocking activated EGFR signaling in tumor cells with this Mab represents an intriguing strategy to minimize normal tissue toxicity [41]. Phase I clinical trial testing with mAB806 (ABT806) has been completed in patients with advanced solid malignancies (NCT01255657) although results have not yet been reported [42].
Preclinical studies have also investigated combinations of radiation and mAbs or TKIs that target EGFR in NSCLC, breast adenocarcinoma , and glioblastoma [40, 43–45]. Effects on in vitro intrinsic radiosensitivity as determined by clonogenic survival assays have been modest but consistent in most instances [2]. In vivo results from the combination of radiation and EGFR inhibition have typically been more striking with concurrent treatment, resulting in greater than additive effects on tumor growth delay [2]. In vivo radiosensitization has been achieved with both single fractions of radiation as well as the more clinically relevant fractionated radiation schedules [7, 46]. For example, treatment of tumor xenografts with gefitinib [47, 48] in combination with radiation resulted in inhibition of tumor growth that was greater than either modality alone. The discrepancy between in vitro and in vivo results has been hypothesized to be related to several different mechanisms that would only be apparent in vivo including inhibition of angiogenesis and reduction in tumor cell invasion [2].
Secondary to the promising preclinical results in the aforementioned paragraphs, numerous clinical trials have been designed evaluating the efficacy of combining EGFR inhibitors with radiation [49]. Perhaps the most notable of these trials was a phase III multicentered randomized controlled trial with 424 patients with locoregionally advanced HNSCC [50]. The trial compared treatment with radiotherapy alone to radiotherapy plus cetuximab . The results were striking and showed an increase in overall survival (OS) from 29.3 months with radiotherapy alone to 49.0 months with the combination of radiotherapy and cetuximab (hazard ratio for death 0.73; P = 0.03). Local control rates were also significantly improved with the addition of cetuximab to radiotherapy (50 % vs. 41 % in the radiotherapy alone arm).
Building upon the Bonner et al. study, RTOG 0522 was designed to answer the question as to whether the addition of cetuximab to cisplatin-based standard chemoradiotherapy (CRT) improved outcomes [51]. This phase III clinical trial randomized patients to concurrent CRT (cisplatin + radiotherapy) alone or with cetuximab in patients with stage III/IV HNSCC. The results of the study showed no difference in OS or PFS with the addition of cetuximab to standard cisplatin-based CRT. However the critical unanswered question is whether cetuximab could replace cisplatin as a radiosensitizing agent for definitive CRT of locoregionally advanced HNSCC. RTOG 1016 was designed to answer this question in a subset of HPV-positive HNSCC [42] and randomizes patients with oropharyngeal cancer to CRT with cisplatin or cetuximab . This trial began recruiting in 2011 and outcomes are pending.
The combined results of the Bonner et al. trials, the preclinical data suggesting that EGFR is a target for radiosensitization , and data showing that the majority of NSCLCs overexpress EGFR led to the development of a 2 × 2 phase III trial in NSCLC evaluating the use of cetuximab and radiation dose escalation up to 74 Gy [52]. The results of this study, however, were disappointing and showed that addition of cetuximab to chemoradiotherapy in patients with locally advanced NSCLC did not affect patient survival. Why did cetuximab fail to radiosensitize NSCLC? The most likely explanation is that the radiosensitizing effect of EGFR inhibition was not additive with chemotherapy. Alternative explanations include the possibility that tumors from this primary site either contain parallel signaling mechanisms that compensate for EGFR inhibition or that EGFR is not a primary driver of cell survival.
The results of the RTOG 0617 and other negative clinical trials combining EGFR targeting with radiation/chemotherapies raise several important questions about how to advance this treatment strategy. The most important of these is how patients that respond to EGFR inhibition in combination with radiation can best be identified prior to treatment. This concept is currently undergoing extensive evaluations in both the laboratory and the clinic [2]. For example, it has been suggested that p16+ HNSCC are more sensitive to the combination of cetuximab and radiation [53]. In contrast, and somewhat surprisingly, EGFR expression has not shown to correlate to response to combination chemotherapy and cetuximab [54]. In fact responses to EGFR inhibition have been shown with a lack of EGFR staining by immunohistochemistry (IHC) [55], confirming that identification of mechanistic biomarkers will be valuable for directing future approaches for EGFR targeting and radiosensitization .
VEGF/VEGFR
Angiogenesis is a hallmark of tumor progression and metastasis, and the VEGF growth factor and its receptors play critical roles in the regulation of angiogenesis [56]. The VEGF family of proteins consists of VEGF A–E and placenta growth factor (PLGF) 1–2. VEGFA is the most abundant of the VEGF proteins and exerts its effects primarily by binding to VEGFR-1 and VEGFR-2 [56, 57]. Like the EGFR, both transmembrane RTKs are stimulated by ligands and undergo dimerization, autophosphorylation of its intracellular tyrosine residues, and initiation of downstream signaling [56]. These receptors exist primarily on vascular endothelial cells [56, 57]. VEGFR-1 is thought to be involved in vascular system development during angiogenesis, whereas VEGFR-2 is the primary mediator of the angiogenic, mitogenic, and vascular permeability-enhancing effects of VEGF. VEGFR-2 signals downstream via PI3K/AKT/mTOR and the RAS/MAPK pathways to enhance endothelial cell proliferation and survival [57].
VEGF is overexpressed in many solid tumors [57]. This increased expression has been shown to correlate with worse PFS and OS [56, 58]. As such anti-VEGF therapy has garnered significant interest as a cancer therapy, and development of bevacizumab , a humanized monoclonal antibody, is directed against VEGF that prevents its binding to VEGFR-1 and VEGFR-2 [59].
It was initially thought that antiangiogenic therapy would impair the effects of ionizing radiation by the induction of tumor hypoxia, as oxygen is thought to be critical to the formation of free radicals that cause DNA double-strand breaks and cell death [60]. However, early studies by Teicher et al. showed that this might not be true for all tumors as antiangiogenic therapy with the angiogenesis inhibitor TNP-470 and minocycline actually improved tumor oxygenation and the antitumor effects of radiotherapy [61]. Furthermore the interaction with EGFR signaling, which potentiates production of VEGF [62], suggests that enhanced angiogenesis is a mechanism for both tumor and vessel radioresistance [62]. Because of the increase in oxygenation with antiangiogenic therapies and data showing enhancement of VEGF levels by RT, it was postulated that strategies targeting angiogenesis might augment the radiation response.
In the first preclinical study of a targeted antiangiogenic therapy with radiation, it was shown that angiostatin , a natural product that inhibits angiogenesis, enhanced the effects of radiation on in vivo murine lung cancers as well as human glioblastoma , squamous cell carcinoma , and prostate carcinoma xenografts [63]. Gorski et al. showed that anti-VEGF antibodies in combination with radiation (20 and 40 Gy) in several tumor xenografts (lung carcinoma , squamous cell carcinoma , glioblastoma , and esophageal carcinoma ) caused a greater than additive increase in tumor growth delay than either therapy alone [64]. DC101 an inhibitor of mouse VEGFR-2 has also been used in several preclinical studies to enhance the effects of radiation [65]. Kozin et al. showed that the use of DC101 before, during, and after fractionated radiation therapy decreased the dose of radiation required to control 50 % of tumors locally in 54a (lung carcinoma) and U87 (glioma) xenografts by 1.7- and 1.3-fold, respectively [66].
Several potential mechanisms have been described with regard to anti-VEGF therapies and increased response to radiation. First, it has been suggested that anti-VEGF therapy increases the radiosensitivity of vascular endothelial cells [64]. Several studies have shown increased apoptosis of vascular endothelial cells with anti-VEGF therapy and radiation [65, 66]. The increased death of endothelial cells then can reduce vascular density and inhibit the formation of new blood vessels causing impaired nutrient delivery to the tumor [65]. Secondly, studies have shown that anti-VEGF agents can renormalize the vasculature causing an increase in tumor oxygenation and hence an increase in tumor radiosensitivity [67, 68].
Secondary to the promising preclinical findings mentioned above, clinical trials have been performed with anti-VEGF therapy both as monotherapy and in combination with radiation. Several phase I/II clinical trials have been published showing promising results in many tumor types (e.g., glioblastoma , rectal cancer , and HNSCC) [69]. These early clinical trials in patients with glioblastoma led to the development of two phase III clinical trials. The RTOG 0825 was a phase III double-blind randomized controlled trial comparing conventional concurrent chemoradiation and adjuvant temozolomide plus bevacizumab vs. conventional concurrent chemoradiation and adjuvant temozolomide in patients with newly diagnosed glioblastoma [70]. The data showed that there was no increase in OS with the addition of bevacizumab to standard therapy even though there was a trend toward increased PFS (HR, 0.79; 95 % CI, 0.66 to 0.94; P = 0.007). A similar study, AVAglio (Avastin in glioblastoma), was a phase III study that evaluated the efficacy of adding Avastin (bevacizumab ) to standard chemoradiation and adjuvant temozolomide in patients with newly diagnosed glioblastoma [71]. After surgery or biopsy, patients were randomized to receive concurrent radiation and temozolomide plus either Avastin or placebo. After the completion of six cycles of maintenance temozolomide and Avastin or placebo, the patients continued on Avastin or placebo until disease progression or unacceptable side effects are presented. OS was nearly identical between the two arms. The improvement in PFS (10.6 months with Avastin vs. 6.2 months with placebo; HR 0.64; 95 % CI, 0.55–0.74; P < 0.001) observed in this study reflects the drug’s clinical effectiveness for targeting angiogenesis without enhancing the radiosensitivity of glioblastoma tumor cells.
The disappointing results of these clinical trials in GBM suggest that the rationale for radiosensitization must be reevaluated. Chief among these concerns is the hypothesis that VEGFR inhibition does not increase hypoxia in human tumors as this effect could counteract the combination of antiangiogenic therapy with radiation. Additionally biomarkers and patient selection may also provide a way to identify patients most likely to benefit from anti-VEGF agents. We also know from preclinical results that treatment combinations with anti-VEGF agents and radiation are treatment dose dependent [72]. This emphasizes careful consideration and understanding of the clinical design of combinations of radiation and anti-VEGF agents. In addition, similar to anti-EGFR agents, patients being treated with anti-VEGF/VEGFR agents experience resistance to therapy [72]. In patients with glioblastoma who experienced clinical progression on cediranib (a potent TKI of VEGFRs), significant increases in plasma bFGF and stromal cell-derived growth factor (SDF1a) were noted [73]. This is in agreement with preclinical models where cross talk between many angiogenic factors including VEGF, PDGF, angiopoietins, ephrin, and Notch has been shown [56, 74]. Thus although inhibition of a single factor may not be sufficient to fully inhibit angiogenesis in all patients, study of rationale combinations of anti-VEGF therapy with other targeted agents in preclinical models may provide valuable insights for combining RT with targeting of angiogenesis .
c-Met
c-Met, also known as the hepatocyte growth factor (HGF) receptor , is a 170-KD transmembrane receptor tyrosine kinase that plays an important role in tumorigenesis and metastasis [75]. Like other RTKs , ligand binding activated receptor activity through dimerization and phosphorylation of intracellular tyrosine kinase domains [76]. Downstream signaling occurs through many of the previously mentioned oncogenic signaling pathways including PI3K/AKT/mTOR, RAS/MAPK, and JAK/STAT [77, 78].
HGF was originally identified as a cytokine that caused the dissociation of colonies of cells into single cells [79] and is a pro-migratory ligand that accumulates in the extracellular matrix and is linked to tumor cell invasion. HGF also promotes epithelial-mesenchymal transition (EMT) [79–82], which in turn causes further increases in tumor cell migration, invasion, and angiogenesis [77].
HGF/c-MET autocrine ligand signaling is aberrantly activated in a number of different cancers including breast, glioma, NSCLC, SCLC, and colon cancer [83–88]. Increased production of HGF by both cancer cells and the surrounding stroma as well as gene amplification and overexpression of c-Met has been described as mechanisms for activating this autocrine loop. Increased production/upregulation of the HGF/c-Met axis has also been shown to be a negative prognostic indicator [77, 89, 90]. For example, increased expression of HGF and c-Met in colon cancer is associated with worse disease stage [91], lymph node metastasis [91], and decreases in PFS and OS [86].
c-Met activation and signaling has been linked to resistance to both DNA-damaging chemotherapies and ionizing radiation [77]. One of the earliest studies to link HGF/c-Met and resistance to DNA-damaging therapies was done by Fan et al. [92]. This study showed that pretreating breast cancer cells with HGF decreased DNA fragmentation induced by DNA-damaging agents. In a further study, they showed this effect to be mediated by c-Met through the PI3K/AKT pathway [93]. In clinical studies increased c-Met expression has been shown to be an independent predictor of local failure in patients undergoing definitive radiation for SCC of the oropharynx [94].
Preclinical studies have explored the relationship between radiation and c-Met signaling. De Bacco et al. showed that irradiation induced c-Met expression in a variety of cell lines [95]. Furthermore they found that inhibition of c-Met activity with the small molecule tyrosine kinase inhibitors PHA665752 (or JNJ-38877605) sensitized glioma and breast cancer cells to irradiation in vitro and in tumor xenograft model systems [95]. Increased c-Met expression/activation after radiation has been reported in pancreatic cancer, glioblastoma, and neuroblastoma model systems [96–98], and Chu et al. reported that in glioblastoma cells, radiation-induced HGF secretion leads to activation of c-Met signaling in glioma cell lines [97].
Based upon the above observations several groups have begun to define the role of HGF/c-Met in mediating cell survival after exposure to ionizing radiation. Welsh et.al showed that inhibition of c-Met with siRNA and the small molecule inhibitor MP470 can radiosensitize glioma cells to radiation in vitro and in vivo [99]. In these studies, radiation-induced DNA damage repair via a decrease in Rad51 expression after irradiation was implicated as the mechanism for radiosensitization . In gastric carcinoma cells, inhibition of c-Met was shown to decrease phosphorylation of ATR and checkpoint kinase 1 (CHK1) [100]. Similar results demonstrating radiosensitization have been shown in other glioblastoma xenograft models as well as in vitro and in vivo models of prostate cancer, thyroid cancer, and NSCLC [101–105].
A number of different inhibitors of the HGF/c-Met signaling axis are available for clinical use [77, 106]. These include anti-HGF antibodies (ficlatuzumab , rilotumumab , and TAK-701 ), anti-Met antibodies (onartuzumab ), and small molecule tyrosine kinase inhibitors (cabozantinib , foretinib , and tivantinib ). Several of these molecules have been combined with other targeted agents including anti-EGFR inhibitors [77].
To date only one clinical trial of radiation and c-Met inhibition has been performed. This was a phase 1 safety trial of cabozantinib with temozolomide and radiation in newly diagnosed glioblastoma patients. The study closed in 2013 and the results have not been reported at the time of this publication [42]. Given the aforementioned preclinical and clinical data, it is logical to further explore the combination of radiation and c-Met inhibition with the goal of testing whether inhibition of Met signaling can enhance the effects of radiation therapy in malignant tumors .
Other RTKs
There are several other RTKs that have been studied with regard to their role in the radioresponse, however, to a lesser degree than the previously described receptors. One such studied RTK is the insulin-like growth factor-type 1 receptor (IGF-1R) . The IGF family proteins are the primary ligand for IGF-1R [107]. Their binding acts similarly to the other RTKs discussed above [108]. IGF-1R signaling has been linked to malignant transformation, cellular proliferation, cell survival and differentiation [109], as well as increased local recurrence after RT [110].
With regard to regulation of the radiation response, several preclinical studies have been performed showing radiosensitization both in vitro and in vivo. Riesterer et al. showed that the use of A12, an anti-IGF-1R antibody, caused radiosensitization of HNSCC cell lines in vitro via the clonogenic survival assay as well as in vivo as measured by tumor growth delay [111]. Allen et al. published similar results with the combination of A12 and radiation in H226 lung cancer xenografts [112]. Recent data by Chitnis et al. reports IGF-1R inhibition by AZ12253801, a selective IGF-1R tyrosine kinase inhibitor that radiosensitizes tumor cell lines via an inhibition of both HR and NHEJ [113].
More recently several studies have begun to define the use of IGF-1R inhibitors in combination with radiation and EGFR blockade. The rationale for these studies lies in data showing cross talk between the EGFR and IGF-1R pathways at multiple levels [114, 115]. In fact, EGFR inhibition has been shown to cause increased response to IGF-1R ligands [114, 115]. Li et al. demonstrated that co-inhibition of EGFR and IGF-1R using specific small molecule tyrosine kinase inhibitors to both receptors caused synergistic radiosensitization in breast cancer cell in vitro and in tumor xenografts [116].
HER2 (or erbB2) is an RTK that has no known soluble ligand. However, it exerts its actions by formation of heterodimers with the other ErbB family members, notably EGFR . HER2 overexpression has been noted in approximately 30 % of breast cancers [117] and 20 % of gastroesophageal (GE) and gastric cancers [118]. Trastuzumab , a monoclonal antibody to the external domain of HER2, has been approved for clinical use in metastatic breast cancer and shows activity in preclinical models as well [119]. With regard to the role of HER2 in regulating radiosensitivity, much less is known, with only a few reports combining radiation with specific anti-HER2 therapies . One such study by Pietras et al. showed that trastuzumab treatment radiosensitized the breast cancer cell line MCF7 in vitro and in tumor xenografts only under conditions of HER2 overexpression [120]. Instead, most studies examining the role of Her2 in the radiation response have focused on the use of lapatinib a dual EGFR and Her2 inhibitor. Using lapatinib , several groups have shown an increase in radiosensitivity [121–123]. For example, Sambade et.al demonstrated that the effects of lapatinib plus radiation on tumor growth of HER2+/EGFR+ breast cancer xenografts were greater than additive of either therapy alone [121].
Although there is a paucity of preclinical data with regard to the combination of radiation and anti-HER2 therapies, there have been several clinical trials completed combining the two treatments. The Brown University Oncology Group performed a pilot study of trastuzumab in addition to chemoradiation in patients with HER2+ locally advanced esophageal adenocarcinoma [124]. Despite the patients’ advanced burden of disease, a 3-year OS of 47 % was observed with no increase in adverse events. This study led to the development of RTOG 1010 in which patients with HER2+ locally advanced esophageal adenocarcinoma and GE junction tumors are randomized to chemoradiation plus concurrent and maintenance trastuzumab or chemoradiation [119]. This trial is still open to accrual [42]. In breast cancer, several large clinical trials including the NSABP B-31 and NCCTG N9831 trials have been completed [42]. These trials have compared the addition of trastuzumab to chemotherapy in node-positive or high-risk node-negative nonmetastatic, operable breast cancer patients [125]. Approximately 70 % of patients in both studies underwent adjuvant radiotherapy concurrently with trastuzumab. DFS (P < 0.001; stratified HR, 0.52; 95 % CI, 0.45 to 0.60) and OS (P < 0.001; stratified HR, 0.61; 95 % CI, 0.50–0.75) were significantly increased with the addition of trastuzumab. While this study was not directly comparing the effect of adding trastuzumab to adjuvant radiotherapy, some of the effects seen may have been due to this addition.
The fibroblast growth factor (FGF) pathway has also been studied with regard to its role in regulation of the cellular response to radiation. The FGFs mediate their biological effects through the FGF receptors (FGFRs) . The four known FGFRs include FGFR-1, FGFR-2, FGFR-3, and FGFR-4 [125, 126]. Their activation is controlled by a unique combination of ligand (FGFs) binding as well as heparin sulfate glycosaminoglycan cofactors [127]. The FGF/FGFR signaling axis has a well-documented role in cancer [126]. Activating mutations, receptor overexpression , and alternative splicing have been shown to augment tumorigenesis in a variety of malignancies [126, 128–132]. Expression of FGFR-1 is a known predictor of poor overall survival and shorter time to progression in patients with glioblastoma [132, 133].
Several early reports began to define the role of the FGF/FGFR axis in regulating cellular survival after radiation [130, 133–135]. Fuks et al. showed that basal FGF (bFGF or FGF2) protected endothelial cell from radiation-induced apoptosis and that administration of bFGF to mice protected against the development of fatal radiation pneumonitis [134]. Other studies showed that expression of FGF2 in human tumor cell lines led to an increase in their relative radioresistance via the small GTPase RhoB [136]. A recent study by Ader et al. used an allosteric FGFR small molecule inhibitor, SSR128129E, to determine the effects of FGFR inhibition on glioma cell radiosensitivity [137]. They showed that inhibition of FGFR signaling enhanced in vitro radiosensitivity of two glioma cell lines via the clonogenic survival assay. Additionally the combination of radiation and SSR128129E significantly enhanced neurologic sign-free survival of mice bearing orthotopic glioma xenografts . Furthermore, Cazet et al. showed that disruption of glycosylation via inhibition of mannose phosphate isomerase inhibited FGFR signaling and enhanced radiosensitivity of glioma cell lines in vitro [133]. The preclinical results are promising and suggest further investigation into the role of FGF/FGFR signaling in regulating the cellular radioresponse both preclinically and clinically.
Conclusion
Significant progress has been made toward the understanding of receptor tyrosine kinase signaling in radiotherapy. The extensive body of literature reviewed above with regard to EGFR , VEGF/VEGFR , c-MET, IGF-1R, and HER2 illustrates this progress. These findings underscore the importance of the rational translation of preclinical data to the clinical setting. Perhaps the best example of this success is shown by the Bonner et al. showing substantial overall survival benefit with the addition of cetuximab to radiation therapy in HNSCC patients [50].
These successes in the preclinical and clinical settings are not without their limitation. First of all, resistance to these therapies is common [27, 119, 138]. As discussed above, the mechanisms of resistance are complicated and can possibly vary from tumor to tumor, and thus we are only beginning to understand the mechanisms of resistance. This understanding will allow us to pursue logical combinations of targeted agents in combinations with radiotherapy both preclinically and clinically. Secondly, it appears that with many of the agents that target RTKs , only a subset of tumors actually responds to a given therapy. This fact underscores the importance of being able to prospectively select patients for a given therapy. As illustrated above, this work has begun but has proved to be challenging and will require further investigation. Thirdly, in many of the clinical trials with agents targeting RTK pathways, there was a lack of true target engagement [2]. Being able to determine whether an agent is clearly inhibiting its target in the tumor and actually having an effect on downstream signaling is paramount to being able to judge success or failure in the clinic. While this may be challenging, it is of utmost importance to ensure proper interpretation of results.
Importantly an understanding of which molecular subtypes of tumors will respond to the combination of radiation and RTK inhibition will be of considerable significance. This has been an area of considerable interest with regard to inhibitors of RTKs as monotherapies [2, 27]. For instance, in lung carcinoma , it has been demonstrated that tumors that harbor KRAS mutations are resistant to EGFR inhibition [139]. Similarly PTEN deletion in glioblastoma patients causes resistance to EGFR-directed therapeutics. As both KRAS mutations and PTEN cause activation of signaling downstream of RTKs, it is rational to expect these mutations to confer resistance to inhibitors upstream molecules. A recent study by Bennett et al. extended these results to the combination of radiation and inhibition of RTK signaling via aclacinomycin (Acm) treatment [140]. They demonstrated that Acm was only effective as a radiosensitizer when used on cell lines that were EGFR dependent but not on cell lines that harbored KRAS mutations (EGFR independent) [141]. These results underscore the importance of choosing tumors with molecular characteristics that will be expected to respond to RTK-targeted therapies. As such future studies aimed at determining molecular signatures of responsive tumors will bear relevance to molecular radiation oncology.
While the mechanism of action of how these agents interact with radiation is beginning to be elucidated, much has yet to be learned. A mechanistic understanding of this interaction will allow for differing treatment schedules and rationale combinations with other therapies. Additionally, understanding the mechanisms of radiosensitization may allow us to exploit certain tumors based upon their specific genotypes or pathway alterations. As such continued investigation into all of the above RTKs should continue to provide a wealth of knowledge that will ultimately be able to benefit patients with many different types of tumors.
References
1.
Druker BJ, Sawyers CL, Kantarjian H, Resta DJ, Reese SF, Ford JM, Capdeville R, Talpaz M (2001) Activity of a specific inhibitor of the BCR-ABL tyrosine kinase in the blast crisis of chronic myeloid leukemia and acute lymphoblastic leukemia with the Philadelphia chromosome. N Engl J Med 344(14):1038–1042. doi:10.1056/NEJM200104053441402 PubMed
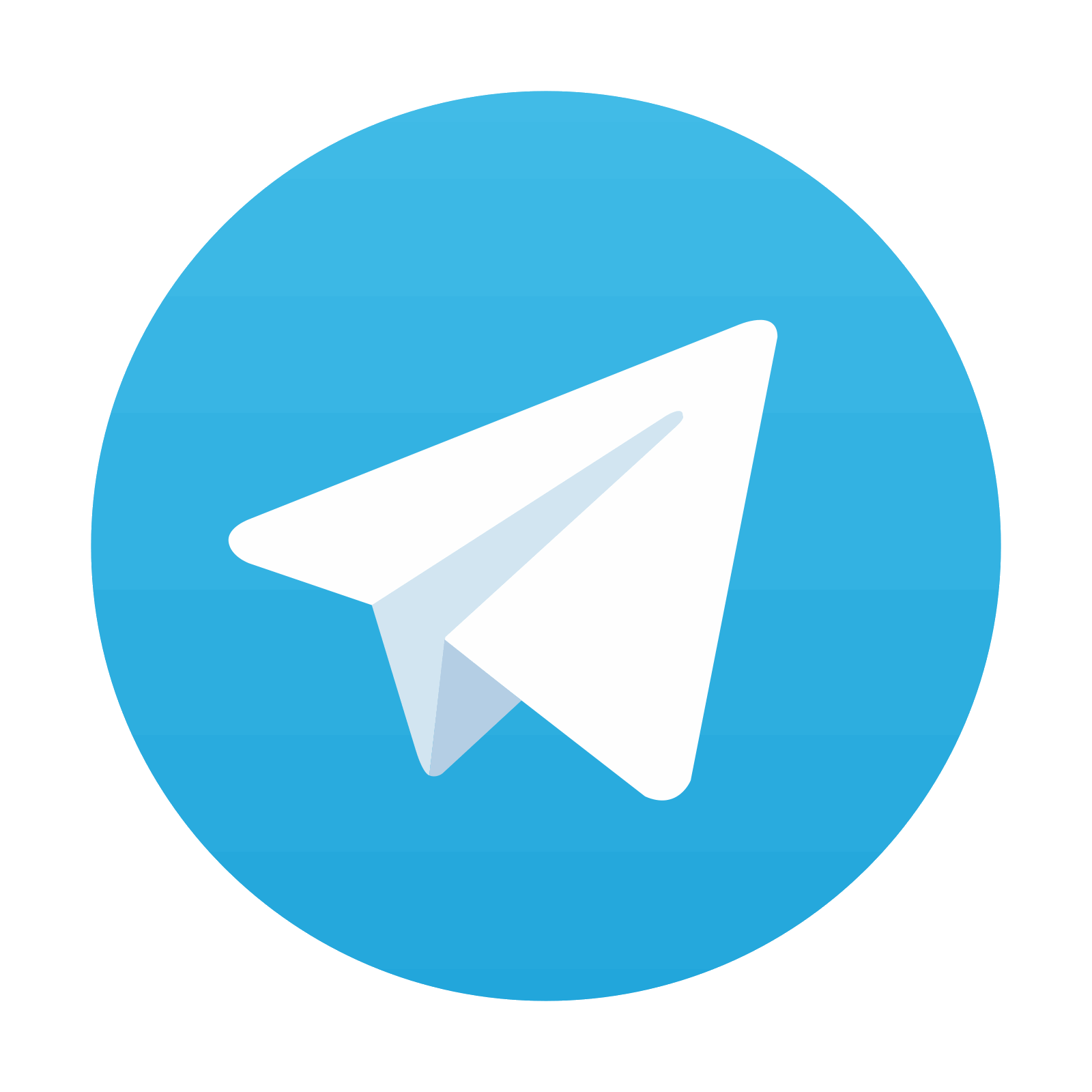
Stay updated, free articles. Join our Telegram channel
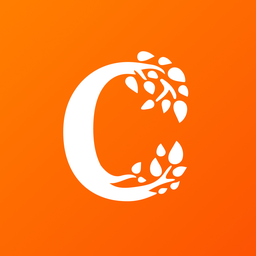
Full access? Get Clinical Tree
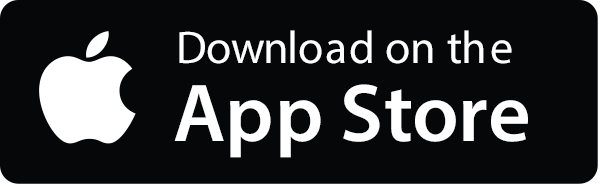
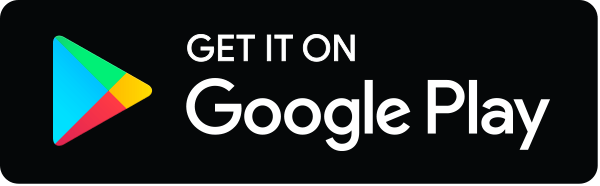
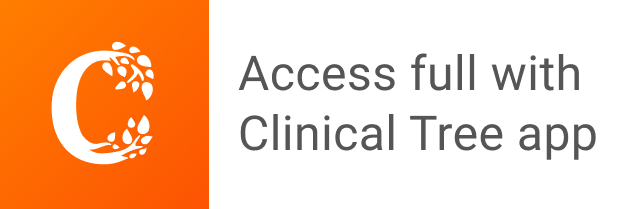