© Springer International Publishing Switzerland 2017
Philip J. Tofilon and Kevin Camphausen (eds.)Increasing the Therapeutic Ratio of RadiotherapyCancer Drug Discovery and Development10.1007/978-3-319-40854-5_66. Remodeling the Irradiated Tumor Microenvironment: The Fifth R of Radiobiology?
(1)
Department of Radiation Oncology, Helen Diller Family Comprehensive Cancer Center, University of California, 2340 Sutter Street, San Francisco, CA 94147, USA
Abstract
With the recognition that the host and tumor are inextricably intertwined as a malignant system, the tumor microenvironment (TME) is now considered to be an important target in cancer therapy. A long-standing objective to improve the therapeutic ratio of radiotherapy has been to manipulate microenvironmental factors that impede cancer cell radiosensitivity, most notably hypoxia. A more recent idea is to eradicate or reeducate the components of TME that support and sustain cancer in order to better control the local tumor and prevent metastatic disease. The TME includes the vasculature, stromal cells, and immune cells, each of which is locally corrupted by the presence of cancer, which itself influences distant tissue and cell behaviors. The biology of the irradiated TME provides robust targets to augment local tumor control and intersect with immunological mechanisms that can eliminate distant disease. Transforming growth factor β (TGFβ) is an example of a critical mediator of the irradiated TME. TGFβ inhibition in the context of radiotherapy is predicated on understanding its mechanistic opposition to therapeutic benefit. Therapeutic strategies to biologically augment radiotherapy by preventing the reestablishment of a functional TME could motivate the addition to the classic “Rs” of radiation biology in oncology: repair, reoxygenation, repopulation, redistribution, and now, remodeling the TME.
Keywords
Tumor microenvironmentTGFβExtracellular matrixRadiotherapyCancerIntroduction
At least one course of radiation therapy is prescribed to more than half of all patients with solid tumors. The therapeutic efficacy of radiation is generally considered to be mediated by its capacity to directly kill cancer cells, encouraging dose escalation strategies and combinations with chemotherapy to enhance its antitumor effect. The basis for radiation therapy is often described in terms of the 4 Rs of radiobiology: repair, reoxygenation, repopulation, and redistribution. Double-strand DNA breaks cause a molecular response that results in cell cycle arrest and repair or cell death by diverse processes ranging from apoptosis to senescence [1]. Accordingly, efforts to understand and improve the therapeutic efficacy of radiotherapy have largely concentrated on the molecular mechanisms of DNA damage recognition and repair.
When cancer is treated with radiation, death of tumor cells is coupled with tumor microenvironment (TME) changes that accompany tumor regression. The radiation response of tumor cells has been far better characterized than that of the TME , with the major exception of hypoxia which is a critical barrier to tumor control [2]. Radiation oncology principles thus incorporate the vascular response as a concomitant therapeutic target and resultant effects of radiation on hypoxia (i.e., reoxygenation ). However, it is becoming increasingly clear that additional aspects of the TME can contribute to tumor control and even determine radiation sensitivity.
The growing appreciation that TME complexity is inextricably intertwined with tumor growth, metastasis, and response to therapy has engendered clinical strategies to target the TME [3]. Here, we focus on the potential of knowledge about the TME response to radiotherapy . Understanding TME dynamics in response to radiation provides a view of the tumor as an adaptive “organ.” This area of investigation is in an exponential growth phase as evidenced by the number of research papers on which radiotherapy and microenvironment are keywords, from 11 in 2000 to 250 in 2015, but is still relatively understudied, particularly as a function of fractionation. One might thus propose the addition of remodeling of the TME to the “Rs” of radiobiology.
The Tumor Microenvironment
The cellular component of the TME is comprised of inflammatory cells, innate and adaptive immune cells, including natural killer cells, T cells, and B cells, and cancer-associated fibroblasts [4]. These cells collectively create a stromal environment that promotes tumor progression by providing growth factors, pro-angiogenic factors, proteases, and adhesion molecules that facilitate tumor cell proliferation, angiogenesis, invasion, and metastasis [5, 6]. The dynamic changes in the TME also provides a selective pressure for tumor cell variants that may promote genomic instability, genomic heterogeneity, and epigenetic alteration [7].
In order for cancer to evolve from early dysplastic lesions into invasive cancer, further changes in both the epithelium and the stroma are required to establish the tumor. When initiated cells activate oncogenic signaling pathways that promote growth, motility, and resistance to apoptosis, they also activate the stroma, leading to further recruitment of inflammatory cells and reprogramming of the stromal niche [8]. For example, many of the key downstream targets of mutant Kras are cytokines and chemokines that promote progression to dysplasia in a large part through activation and remodeling of stromal cells [9]. Thus, initiated cells begin to “educate” the niche cells to provide greater growth signals and immune suppression. Moreover, these stromal educating signals are not confined simply to the local microenvironment, but are in fact systemic signals. Recent data indicate that as carcinogenesis progresses to the stage of dysplasia , there are systemic signals sent out that lead to profound reprogramming of the bone marrow stroma, with increased production of bone marrow myofibroblasts and recruitment of mesenchymal stem cells into the blood stream and then into the tumor site [10]. The education of the bone marrow by the tumor is likely to be a critical step in cancer progression. The nature of these signals is not fully defined but could include TGFβ, SDF-1, and possibly exosomes. Prior to metastatic spread of cancer , activation and generation of distant “niches ” provide a suitable environment for the growth and spread of the tumor [11, 12]. Early-stage tumors are able to generate these “pre-metastatic niches ” in a systemic fashion, and these niches are likely a major factor determining metastatic spread [13]. Indeed, the ability to metastasize is probably acquired early on by many cancer cells, and individual tumor cells are likely released frequently into the circulation. However, in the absence of suitable niche cells, these cancer may remain localized for years.
With respect to treatment of established cancer , emerging evidence supports the idea that the stromal niche contributes substantially to chemoresistance and to the inability to eradicate cancer [14]. This could be due in part to the role of stroma as a barrier to drug delivery, via high interstitial pressure [15], but likely the stroma also contributes positively to signaling pathways that sustain cancer cells and/or inhibit apoptosis and cell death. Thus, additional efforts to understand the TME, and to target key pathways or cellular components, may be important to improve therapies for many solid tumors.
Most epithelial-derived tumors are characterized by the recruitment of mesenchymal-derived stromal cells , including intratumoral and peritumoral fibroblasts , often designated as cancer-associated fibroblasts (CAF) , that mediate tumor growth, angiogenesis , invasion, and metastasis [16, 17]. CAF are often described as being in an “activated” state, as might occur in wound healing, which is phenotypically distinct from normal fibroblasts. Although their heterogeneity is yet to be fully explored, a subset of CAF has characteristics similar to myofibroblasts based, for example, on expression of α-smooth muscle actin. The mechanism of fibroblast activation in cancer is not completely understood. In adult tissues, differentiation from resident stromal fibroblasts into activated myofibroblasts occurs through paracrine signaling by TGFβ generated by damaged or inflamed tissues [18–20]. Both TGFβ and interleukin-1β induce differentiation of quiescent fibroblasts into activated myofibroblasts, but TGFβ is considered the predominate inducer [21]. Radiation-induced TGFβ-mediated activation of fibroblasts is evident in culture [22] and may occur in tumors. The origins of activated fibroblasts in tumors are also incompletely described. Although the majority of CAF are thought to arise from the activation of resident fibroblasts, activated fibroblasts can also originate from pericytes, vascular smooth muscle cells, bone marrow-derived mesenchymal cells, and epithelial to mesenchymal cell transition [23].
Prognostic significance of CAF-associated gene-expression signature has been reported in multiple independent cohorts of non-small cell lung cancer (NSCLC) patients [24]. When compared with normal fibroblasts, CAF significantly increase the invasiveness of co-cultured lung cancer cells and enhance tumorigenicity in vivo. Genes differentially expressed by CAF compared to normal fibroblasts include signal transduction, response to stress, cell adhesion, and angiogenesis [24].
The ability of tumor cells to induce new blood vessel formation is essential for progressive tumor growth and blood-borne metastasis . Angiogenesis is the formation of new capillaries from pre-existing vessels which is of fundamental importance in development, normal organ maintenance, and homeostasis . Tumor angiogenesis relies on many of the same processes as those involved in physiological angiogenesis. Ischemia and hypoxic conditions, which initiate a cascade of highly coordinated cellular functions resulting in the establishment of new blood vessels and oxygen and nutrient supply, are major drivers of both physiological and tumor angiogenesis. This complex process requires interaction between different cell types, the extracellular matrix , and several cytokines and growth factors.
Under physiological conditions, the chain of events leading to changes in cellular function and composition recedes following vascular perfusion. In contrast, during tumor angiogenesis, the angiogenic cascade is persistent and unresolved, fueled in part by tumor-secreted factors and areas of transient tumor hypoxia due to tortuous vasculature. Angiogenesis contributes to the progression of cancer from a dormant in situ lesion to life-threatening invasive disease.
Inflammatory cells are components of the microenvironment of normal tissues and organs, regulating various processes during development, including epithelial growth and branching and clearance of apoptotic cells [25]. The progressive change in both inflammation and antitumor immunity with tumor stage is important in that a balance toward anti-tumor immunity initially suppresses cancer cell growth, but eventually tumor cells escape this control and elicit inflammation that is more conducive to tumor progression [26].
Of particular importance are myeloid-derived suppressor cells (MDSC) which are a heterogeneous population of immature macrophages, granulocytes, and other myeloid cells in early stages of differentiation and have properties similar to those that have been described for M2 macrophages. More MDSC, marked by CD11b+/CD14+/CD15+/CD33+, are present in the peripheral blood of advanced-stage NSCLC patients compared with healthy controls [27]. MDSC oppose the actions of cytotoxic T cells. Cytotoxic T lymphocytes (CTL) , mostly CD8+, are associated with prolonged survival in squamous cell NSCLC [28]. Stromal CD4+ T cell and co-localization of stromal CD8+ T cells and CD4+ T cell are associated with improved NSCLC survival [29, 30]. In a study of stage I compared to III NSCLC, immunosuppressive tumor-infiltrating Foxp3+ Treg cells were associated with increased tumor recurrence [31]. Anti-tumor tumor-associated macrophages (TAM) are classified as M1 phenotype. M1 accumulate intratumorally, whereas pro-tumor M2 phenotype accumulates in the stroma and expresses interleukin-8 (IL-8) and IL-10 and triggers TREM-1 , a receptor expressed on myeloid cells.
IL-8 is an angiogenic factor, and the angiogenic role of TAM in cancer has been shown by correlating macrophage density with intratumor microvessel counts and poor NSCLC patient outcomes [32]. IL-10 is an immunosuppressive cytokine, and its expression by TAM has been observed more commonly in late-stage NSCLC, which correlates with decreased overall survival. Furthermore, increased levels of TREM-1 high TAM in resected specimens was an independent predictor of shorter overall survival in stage I to III NSCLC patients [33].
In late-stage tumor development, TAM and MDSC also activate TGFβ and are classically involved in cancer progression and metastasis [34]. It is likely that TGFβ acts in an autocrine manner to convert TAM from an M1 to M2 phenotype and that TAM contribute to the general immunosuppressive TME in turn by producing large amounts of TGFβ. MDSC are polarized toward immunosuppression that impedes the ongoing immune and inflammatory response to cancer . Such polarized MDSC suppresses T cells and natural killer cells, as well as antigen-presenting cells, abrogating the antitumor immune response. Thus, the presence of MDSC in cancer poses a serious obstacle to therapies that attempt to stimulate antitumor immune responses. The expansion of MDSC population in the circulation of cancer patients may be useful as a biomarker of TGFβ inhibition. One study reports that polymorphisms in TGFβ pathway members are associated with dramatically different median survival times of 45.39 versus 13.55 months and 18.02 versus 5.89 months for high- and low-risk populations of NSCLC patients as a function of chemoradiation and chemotherapy, respectively [35].
A canonical target of TGFβ is the extracellular matrix (ECM) , which is actively remodeled during tumor development and in response to therapy. The composition of the ECM is tissue specific, e.g., collagen rich in epithelial organs in which it provides a “skeleton” to support the parenchyma versus hyaluronic acid rich in the brain supportive of neuronal networks. The ECM composition also differs across cancers and is often a hallmark as in the dense collagen of breast and pancreatic desmoplastic cancers . Matrix metalloproteinases , commonly produced by activated macrophages recruited by TGFβ, degrade ECM components can release TGFβ, VEGF, and other sequestered factors of TME remodeling [36].
TGFβ in Cancer and Radiation Biology
TGFβ biology warrants further exploration in the TME and particularly in the context of therapy. Although TGFβ is a canonical tumor suppressor due to inhibiting epithelial proliferation and inducing apoptosis, loss of response to TGFβ as a growth inhibitor and increased expression of TGFβ is nearly universal in cancer [37]. Increased TGFβ activity in tumors can act in a variety of ways to promote neoplastic progression . Production of TGFβ in malignant cells acts on the host to suppress antitumor immune responses, to enhance extracellular matrix production, and to augment angiogenesis (reviewed in [38]). These activities resemble those induced by TGFβ during wound healing and may create a “permissive” microenvironment that promotes malignant growth by acting on the host.
TGFβ ligands are enriched in the TME where their production by stroma or tumor cells varies according to tumor phenotype [39]. TGFβ activity is controlled by production as a latent complex that requires extracellular modification to initiate ligand binding to ubiquitous receptors; TGFβ activation is efficiently induced by radiation, in part due to the presence of a redox-sensitive motif in the latency-associated peptide (reviewed in [40]). TGFβ is a canonical tumor suppressor due to inhibiting epithelial proliferation and inducing apoptosis [37]. Loss of response to TGFβ as a growth inhibitor and increased expression of TGFβ have been associated with malignant conversion and progression in breast, gastric, endometrial, ovarian, and cervical cancers, as well as glioblastoma and melanoma [37]. Inactivation of the Smad4 gene through homozygous deletion or intragenic mutation occurs frequently in association with malignant progression in pancreatic and colorectal cancer [41]. However, mutation of the TGFβ pathway genes occurs only occasionally in many human cancers. For example, Reiss and colleagues showed that 92 % of more than 500 breast cancers were positive for nuclear, phosphorylated Smad2, indicating activation of the TGFβ pathway [42]. Indeed, many TGFβ transcriptional responses are intact, while cancer cells have escaped the control of proliferation.
The conundrum of why tumors maintain TGFβ expression and signaling when it is an extremely potent growth inhibitor becomes less perplexing when control of the DNA damage response is incorporated. Cancer cells that have high genomic instability fail to progress; indeed invasive breast tumors are more stable than ductal carcinoma in situ [43]. Genomic instability is a less well-recognized consequence of TGFβ loss, yet deletion of Tgfb1 greatly increases genomic instability in murine epithelial cells [44]. Using cultured keratinocytes isolated from newborn Tgfb1 null , heterozygote, and wild-type mice, Yuspa and colleagues found that Tgfb1 null cells spontaneously immortalized more readily than TGFβ competent cells. Compared to wild-type cells, Tgfb1 null cells gave rise to 1000-fold more mutant clones resistant to a chemical, which requires amplification of the dihydrofolate reductase gene. This unexpected phenotype was difficult to place within the pathways controlled by TGFβ.
Following up on this finding, inhibition of TGFβ signaling in non-malignant human epithelial cells using a small-molecule inhibitor of the TGFβ type I receptor kinase increased frequency of centrosome aberrations , chromosomal instability , and spontaneous DNA damage [45]. Moreover, Tgfb1 heterozygote mammary epithelium , which expresses only 10–30 % of wild-type protein levels, exhibits genomic instability at a level comparable to Trp53 heterozygote epithelium . Proteomic profiling of TGFβ-treated mink lung epithelial cells suggests that TGFβ can inhibit DNA repair genes by causing downregulation through ubiquitylation and proteosomal degradation of Rad51, an essential component of the DNA-DSB repair machinery, in a Smad-dependent manner [46]. Rad51 fails to form nuclear foci in TGFβ-treated cells, which results in more DNA fragmentation in response to double-strand breaks compared to untreated controls [46].
Given that epithelial tissues of Tgfb1 null embryos fail to undergo apoptosis or cell cycle arrest in response to high-dose (5Gy) radiation [47], this suggested major defects in the DNA damage pathway; studies in mouse and human epithelial cells demonstrated that TGFβ is necessary for ATM kinase activity elicited by DNA damage [48]. ATM is a phosphoinositide 3-kinase-related serine/threonine kinase that mediates DNA damage responses to initiate, recruit, and activate a complex program of checkpoints for cell cycle, apoptosis, and genomic integrity. Mutations in human ATM lead to ataxia-telangiectasia, a genetic disease characterized by cellular radiosensitivity and high levels of chromosome aberrations [49]. ATM is activated in response to DNA damage caused by IR and, in turn, phosphorylates numerous substrates, thereby modulating cell fate decisions. ATM precisely controls its downstream pathways, often by approaching the same DNA damage process from several different directions, e.g., the cell cycle checkpoints, each of which is governed by several ATM-mediated pathways [50]. Notably, in addition to ATM’s versatility as a protein kinase with numerous substrates, the ATM nexus contains protein kinases that are themselves capable of targeting several downstream effectors simultaneously and thereby concomitantly control subsets of pathways (e.g., the Chk1 and Chk2 kinases). A prototypic example is ATM-mediated phosphorylation and subsequent stabilization of the p53 protein, a major player both in the G1/S cell cycle checkpoint and in damage-induced apoptosis [51].
Tgfb1 depletion by genetic knockout in mouse cells , or inhibition of TGFβ signaling in human cells, compromises ATM kinase activity and autophosphorylation , leading to reduced phosphorylation of critical DNA damage transducers, abrogation of the cell cycle block, and increased radiosensitivity [47, 48]. The ability of exogenous TGFβ to restore these responses indicates that the effect is both cell intrinsic and distal to TGFβ signaling. Radiation induces TGFβ activity in vitro and in vivo both in normal and cancer cells [52–58]. Inhibiting TGFβ signaling in irradiated human cells phenocopies the molecular and cellular consequences of genetic deletion in mouse cells [48]. Consistent with reduced ATM activity, TGFβ genetic deletion in murine cells or signaling inhibition in human cells increases radiosensitivity. The requirement for TGFβ in the genotoxic stress program provides an understudied avenue by which TGFβ acts as a tumor suppressor, whereby its loss would prime genomic instability and cancer progression.
Many TGFβ transcriptional responses are intact, while cancer cells have escaped TGFβ’s control of proliferation. The necessity for TGFβ signaling to maintain genomic stability and the recognition that TGFβ regulates ATM kinase suggests the following: TGFβ acts to initially suppress cancer by ensuring genomic integrity and yet protects malignant cells both by limiting the level of genomic instability and by enabling recovery from DNA damage induced by radiation and other therapies .
TGFβ Inhibition in Radiation Therapy
Studies spanning two decades have identified TGFβ as a key extracellular signal in irradiated tissues and tumors. Among all radiation-induced cytokines , TGFβ arguably elicits the most complex and far-reaching effects in determining outcome. Testing clinically viable TGFβ inhibitors in oncology is motivated by its pleomorphic consequences in cancer , including regulating the DNA damage response, facilitating the mechanism of metastasis , and compromising antitumor immunity [59].
Execution of DNA damage response is compromised by blocking TGFβ activity in the context of RT, which increases both tumor cell radiosensitivity in vitro and tumor growth delay in vivo [60, 61]. Most cancer cell lines treated with a small-molecule inhibitor of TGFβ type I receptor kinase are more radiation sensitive, i.e., 10–70 % less dose is needed to reduce survival to 10 % as measured by clonogenic cell kill. Consistent with deficient ATM activity, ATM autophosphorylation and γH2AX foci formation are decreased. Irradiation of tumors in mice treated with pre-clinical pan-neutralizing TGFβ antibodies shows reduced γH2AX foci and increased tumor growth delay. Inhibiting TGFβ enhances tumor control by radiation in preclinical brain, breast, and lung cancer models. Thus, epithelial tumors appear to rely on TGFβ to effectively mount the DDR, even though most are resistant to TGFβ growth control. These data provide a strong rationale for TGFβ inhibitors as a means to increase tumor response to radiation across a range of cancers [62].
Of particular interest is glioblastoma multiforme (GBM) , which is characterized by a high degree of radioresistance and inevitable local and/or disseminated recurrence. Several clinical trials are underway combining TGFβ inhibition with cancer RT and chemotherapy, including a phase II trial in glioblastoma. The addition of TGFβ inhibitors improves radiation response in preclinical models of GBM [63, 64]. Zhang et al. specifically reported that the addition of the small-molecule inhibitor of TGFβ receptor type I and II kinase, LY2109761, to the current standard of care treatment (radiation and the oral alkylating agent temozolomide) provided benefit. In addition to radiosensitization and tumor growth delay, TGFβ signaling blockade had antiangiogenic and anti-migration effects as well. Mengxian et al. similarly reported radiosensitization, tumor growth delay, and improved survival with the addition of the same small-molecule inhibitor of TGFβ, LY2109761, without combining with temozolomide . They further demonstrated that either TGFβ inhibition or radiation decreased self-renewal of glioma stemlike cells in a neurosphere assay and a greater decrease when these were combined.
Our studies have also added significantly to the growing body of evidence that TGFβ is a therapeutic target in GBM [61]. First, we showed that autocrine TGFβ potentiates an effective molecular DNA damage response and that radiation-induced TGFβ mediates self-renewal signals in glioma-initiating cells (GIC) . Second, the magnitude of radiosensitization (dose-enhancement ratio (DER) ~1.25 by clonogenic assay) is similar to that treated with temozolomide (DER 1.32) [65]. Considering that the addition of temozolomide to radiation therapy in the treatment of GBM was one of the largest breakthroughs in this disease in decades and is now considered the standard of care, radiosensitization of this magnitude reported here must be considered significant, particularly since the radiation sensitivity of GIC increased nearly threefold. Last, GIC produce more TGFβ, which actually enables more effective execution of the DDR and increases self-renewal signals that together ensure survival following radiation exposure [61].
Recent studies in murine triple-negative breast cancer models also suggest that TGFβ inhibition during RT can also drastically reduce metastasis and promote antitumor immunity [66]. It is clear that increased TGFβ in cancer can act in a variety of ways to promote neoplastic progression . Production of TGFβ in malignant cells acts on the host to suppress antitumor immune responses, to enhance extracellular matrix production, and to augment angiogenesis (reviewed in [38]). These activities resemble those induced by TGFβ during wound healing and may create a “permissive” microenvironment that promotes malignant growth by acting on the host. The excellent safety profiles demonstrated in clinical trials [59], as well as the possibility of protection from late normal tissue complications [67], provide further motivation for assessing TGFβ inhibitors as a means to augment response to radiation therapy .
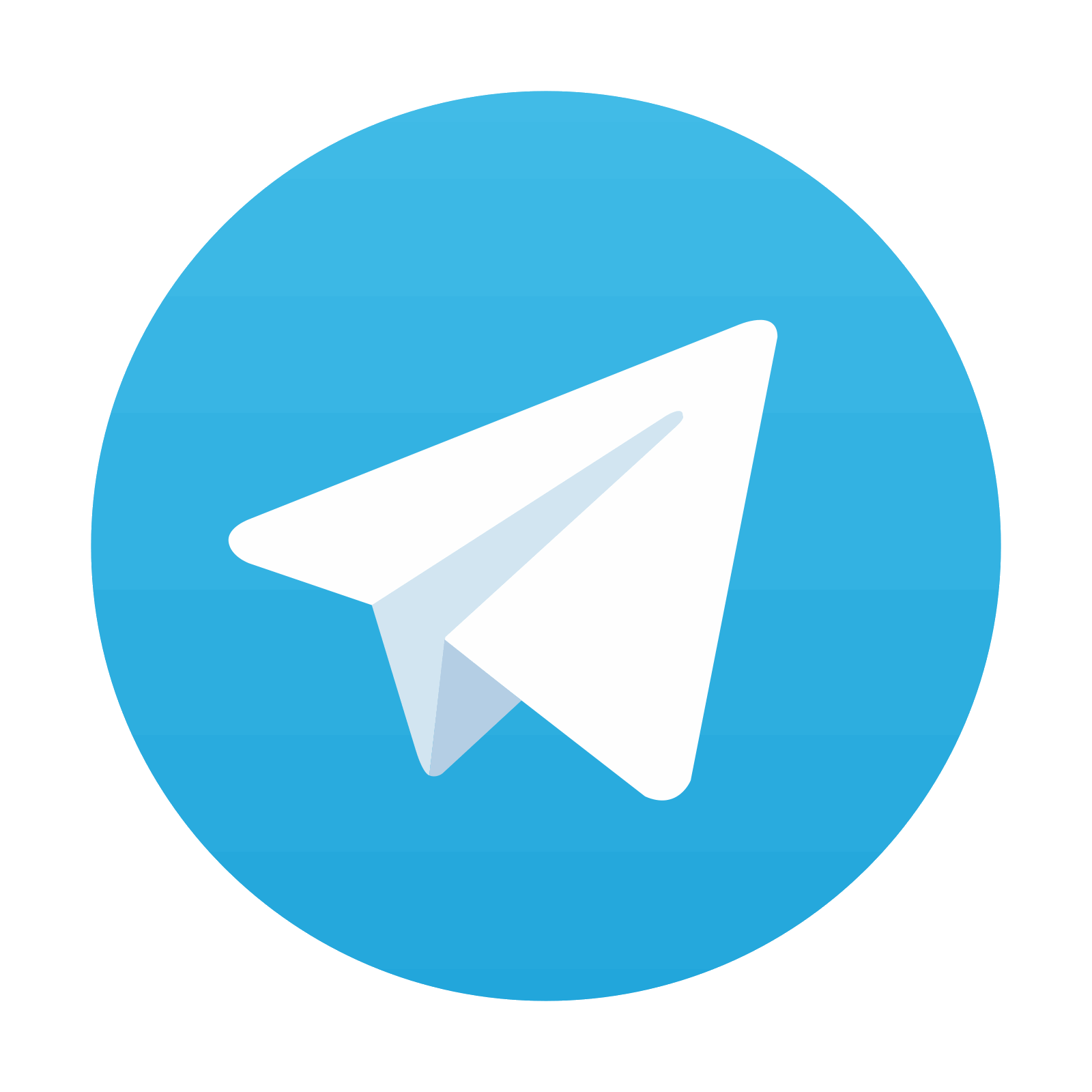
Stay updated, free articles. Join our Telegram channel
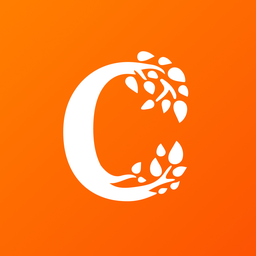
Full access? Get Clinical Tree
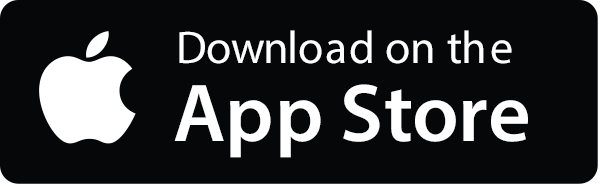
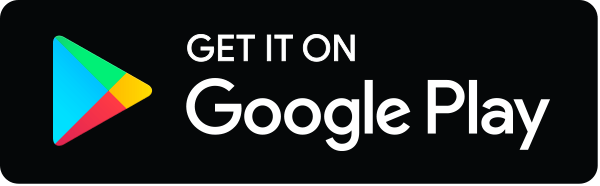