Fig. 8.1
Photomicrographic cross section of human coronary PR, TCFA and FA with varying degrees of luminal stenosis. (a–c) Plaque rupture with mild, moderate and severe luminal stenosis, respectively. Non-occlusive thrombus (Thr) is observed in the microphotograph A, whereas occlusive thrombus is occupying the lumen in the images b and c. (d–f) TCFA with mild, moderate and severe luminal stenosis, respectively. Necrotic core (NC) is covered by a thin fibrous cap, and thrombus is not present in the lumen. (g–i) Stable plaque or FA with mild, moderate and severe luminal stenosis, respectively. The size of necrotic core is relatively small when present, and calcification (Ca++) is frequently seen (Reprinted from Narula et al. [44] with permission from Elsevier)
The thin-cap fibroatheroma is a subtype of fibroatheromas characterised by a large necrotic core (typically >25 % of total plaque volume) that is separated from the lumen by a thin fibrous cap (typically <65 μm). Usually, the fibrous cap tends to be heavily infiltrated with macrophages [31]. This well-characterised lesion is considered to be the plaque subtype most likely to result in plaque rupture and is therefore a key imaging target for both invasive and non-invasive approaches [31].
8.2.5 What Exactly Defines a High-Risk Plaque?
Two distinct plaque morphologies have been implicated in acute coronary events: plaque rupture and plaque erosion. A few cases (2–7 %) are attributable to a third plaque morphology called calcified nodule [37]. It is believed that 60–75 % of acute coronary syndromes are initiated by plaque rupture [38]. Plaque erosion or denudation of the coronary arterial endothelium with an intact fibrous cap is responsible for acute coronary syndromes in most of the remainder cases and is typically observed in young, female smokers [31].
James Muller first introduced the concept of the vulnerable plaque in 1989. Muller categorised ‘hemodynamically insignificant, albeit dangerous lesions as vulnerable plaque’ [39]. Subsequent studies have confirmed the notion that most plaques that cause myocardial infarction are non-flow limiting and therefore missed on antecedent coronary arteriography or myocardial stress testing. Traditionally, the term vulnerable plaque has been used for all plaque types that have been associated with luminal thrombosis [37]; however, recently attention has focused in the thin fibrous cap atheroma as the most common precursor of plaque rupture. In particular virtual histology intravascular ultrasound (VH-IVUS) has been used to identify such plaque in the recent Providing Regional Observations to Study Predictors of Events in the Coronary Tree (PROSPECT) and the VH-IVUS in Vulnerable Atherosclerosis (VIVA) studies [40, 41]. Whilst most of the clinical events in these studies were attributable to TCFAs, what was also clear is the vast majority of these lesions are clinically silent.
Pioneering work over the last two decades has established that plaques that go on to rupture demonstrate several histological features that differentiate them over and beyond the classical intact TCFA. These include a larger necrotic core, higher macrophage infiltration of the fibrous cap and plaque microcalcification [37, 42]. This would suggest that there is an intermediate stage between a thin-cap fibroatheroma and a ruptured plaque, which appears characterised by advanced plaque metabolism [43]. In particular by ongoing macrophage infiltration and cell death within the lipid core, that leads to a self-perpetuating cycle of inflammation, hypoxia angiogenesis and further inflammatory cell infiltration.
How then does calcification fit into this model? Calcification as elsewhere in the body is believed to occur as a healing response to intense inflammation within the necrotic core. Although ultimately advanced areas of macroscopic calcification appear to wall off the thrombogenic contents of this core, thereby imparting stability to the plaque, the very earliest stages of microcalcification are instead consistently associated with increased plaque instability and risk of rupture. In part this may reflect the fact that in the early stages of microcalcification, healing has not yet occurred and the inflamed necrotic environment that predisposes to rupture persists. However, recent data suggests that microcalcification might also directly increase vulnerability, acting as a focal point that intensifies mechanical stresses on the surface of the cap. Whatever the mechanism, microcalcification represents an important additional target for the identification of high-risk plaques.
8.3 Imaging Modalities to Identify High-Risk Plaques
8.3.1 Plaque Morphology with Intravascular Ultrasound
Intravascular ultrasound (IVUS) was one of the earliest invasive techniques to allow imaging of the lumen and vessel wall, and despite the development of novel techniques, IVUS still remains the standard method for assessing plaque burden. This technique also allows us to assess many other structural features within the plaques, including positive remodelling, thrombi, plaque length, lumen narrowing, spotty and macroscopic calcification and the presence of a lipid-rich core [45–49]. Moreover, recent advances making use of ultrasound backscatter (VH-IVUS) provide even more information with respect to plaque morphology and characteristics [50]. Various plaque contents such as calcium, fibrous tissue, fibrofatty tissue and necrotic core can be resolved. Moreover, as discussed, VH-IVUS can classify plaque types such as pathological intimal thickening and thick- and thin-cap fibroatheromas with a high degree of accuracy as compared to ex vivo plaques [50].
VH-IVUS has become widely available and has been the subject of intense investigation, having to date been tested in 3 major prospective clinical studies: Providing Regional Observations to Study Predictors of Events in the Coronary Tree (PROSPECT) [41], VH-IVUS in Vulnerable Atherosclerosis (VIVA) [40] and the European Collaborative Project on Inflammation and Vascular Wall Remodelling in Atherosclerosis (ATHEROREMO-IVUS) study [51]. However, the prospects of identifying patients or indeed plaques that will cause future cardiovascular events using radiofrequency IVUS have been rather disappointing. Indeed, amongst 697 patients enrolled in the PROSPECT study, 595 thin-cap fibroatheromas were identified by IVUS across the cohort, only 26 of these plaques were sites of subsequent events at 3 years and the majority of events were related to rehospitalisation for angina rather than myocardial infarction. Plaque burden greater than 70 %, minimal luminal area less than 4 mm2 and the presence of thin-cap fibroatheromas were thought to be the primary drivers. However, when all these three factors were present in the same lesion, the positive predictive value of an event was only xx%. Consistent with these findings, the VIVA and ATHEROREMO-IVUS studies suggested that whilst identification of thin fibrous cap atheromas identified subsequent MACE, it did not predict future MI per se, and once again the vast majority of these lesions did not result in clinical sequelae.
One of the important limitations of VH-IVUS is its limited axial resolution of 100–150 μm [52], which means that it will tend to overestimate the number of plaques with a thin fibrous cap (traditionally defined by a thickness of <60 μm). This may account for the low event rate associated with VH-IVUS-defined TCFAs.
8.3.2 Assessment of Fibrous Cap Thickness with Optical Coherent Tomography
Optical coherence tomography (OCT) is an imaging modality that provides high-resolution (15–20 μm) tomographic visualisation of the coronary arteries. Although OCT lacks penetration (1–2 mm into the plaque), it is especially good for examining the surface of the plaque, for estimating the thickness of the fibrous cap and for identifying regions of plaque rupture and superimposed thrombus. These high-resolution capabilities make OCT attractive for the identification of high-risk plaques although its poor depth penetration hampers full characterisation of coronary plaque morphology and burden. In particular the potential of OCT to identify TCFAs in vivo is of major potential interest [53], and it has also been suggested that the capability of OCT to measure changes in the fibrous cap thickness could be useful in monitoring the plaque-stabilising effects of statins and other drugs [54, 55]. Future studies investigating whether the identification of plaques with a thin cap on OCT identifies high-risk patients or predicts clinical events are therefore urgently required.
8.3.3 Assessment of Plaque Surface Characteristics with Angioscopy
Angioscopy has demonstrated an association between plaque colour and lipid-rich plaques in patients with MI [56, 57]. Indeed, yellow plaques as defined by quantitative colorimetry have been associated with a large lipid core and a thin fibrous cap. However, the predictive capability of this technology is yet to be defined.
8.3.4 Lipid Core Burden Assessment with Near-Infrared Spectroscopy (NIRS)
NIRS takes advantage of the fact that different organic molecules absorb and scatter NIR light at different degrees and at various wavelengths. The processing of the reflected signal provides information about the chemical composition of different tissues and seems to permit reliable detection of the lipid component. Atherosclerotic plaques, especially lipid-rich plaques, have a specific chemical signature related to cholesterol esters present in lipid cores. Ex vivo studies have demonstrated the feasibility of atherosclerotic lipid-rich plaque detection using near-infrared spectroscopy. A catheter-based NIRS system and a chemometric algorithm for the detection of lipid core plaques were recently developed and prospectively validated in a human coronary artery autopsy study [58]. NIRS data are presented as a ‘chemogram’ representing the probability that a lipid core plaque is present (yellow for high probability, red for low probability). A first-in-human validation study demonstrated the mathematical and statistical similarity of spectra acquired in vivo compared with those obtained in autopsy specimens [59]; the algorithm identified lipid core plaque in 60 % of imaged segments in patients undergoing PCI for stable angina or ACS. NIRS can collect data with rapid acquisition times, avoiding the need to obstruct blood flow. A limitation of NIRS, however, is that it does not create an image of the vessel wall. Thus, no information is provided as to the depth of lipid core plaque nor can it differentiate thin and thick capped atheroma.
The reliability of this technique has been evaluated in histology-based studies, whereas the SPECTroscopic Assessment of Coronary Lipid (SPECTACL) study was the first report to demonstrate the feasibility of a NIRS catheter in the clinical setting. Recently, NIRS has been used to assess changes in plaque composition with the Reduction in Yellow Plaque by Aggressive Lipid-Lowering Therapy (YELLOW) trial [60] using NIRS to assess the short-term effect of intensive medical treatment with rosuvastatin on both plaque burden and composition. PROSPECT II is a natural history, multicenter study, which will assess the ability of intracoronary NIRS to predict adverse clinical events.
8.3.5 Assessment of Calcific Plaque Disease with Coronary Artery Calcium Score
Ever since Agatston et al. described the calculation of the total calcific burden using coronary artery calcium (CAC) scoring in 1990, this method has become a reliable and reproducible non-invasive technique to quantify coronary atherosclerosis [61]. Total CAC score is derived by multiplying individual areas of calcified plaque by a factor derived from the maximal plaque density (CT attenuation) in Hounsfield units and then adding the values obtained for all coronary plaques identified. Subsequent studies have established CT calcium scoring to be one of the most powerful predictors of future cardiovascular events in asymptomatic individuals irrespective of ethnicity [62–66]. Moreover, risk prediction can be further refined by examining the progression of coronary calcification [67].
CT calcium scoring quantifies macroscopic calcification in the coronary arteries. These plaques are believed to be stable, healed and unlikely to rupture. Indeed, it is well established that calcific fibroatheromas and calcified nodules are very rarely responsible for coronary thrombosis [37]. Why then does calcium scoring predict cardiovascular risk related to rupture? The likely explanation is that it provides a surrogate of plaque burden. The more calcified stable plaques you have, the more unstable non-calcified plaques you are also likely to have in addition [68, 69].
8.3.6 Assessment of Low-Attenuation Plaque, Positive Remodelling and Spotty Calcification with Computed Tomography Coronary Angiography (CTCA)
Due to its wide availability and adoption, one of the most important non-invasive techniques for the evaluation of the coronary vasculature is CTCA. Several studies have reported on the correlation between CTCA plaque features with invasive coronary imaging modalities, such as IVUS, IVUS-VH and OCT. Identification of spotty calcification using CTCA has been associated with high-risk plaque type [70]. Furthermore, Motoyama et al. reported that positive remodelling and low-attenuation plaque on CTCA predict a higher likelihood of future plaque rupture [71]. Others have described features such as the ‘napkin ring’ or the ‘signet ring’ sign for identification of plaque vulnerability [72]. However, interpretation of such features on CTCA comes with significant limitations. Suboptimal resolution does not allow precise definition of the vascular boundary, and the extent of positive remodelling may be over- or underestimated. Similarly, low-attenuation plaque is defined based on specific thresholds in the Hounsfield unit measurements which may be influenced by various imaging and technical parameters in regions of non-calcified plaque.
8.3.7 Metabolic Imaging with Positron Emission Tomography-Computed Tomography (PET-CT)
Combined positron emission and computed tomography (PET-CT) is a modern non-invasive imaging technique that combines functional information from PET with the fine anatomical detail provided by CT, allowing the activity of specific pathological processes to be studied within even small structures in the body. This technique has been widely used in the clinical assessment of patients with cancer for many years, resulting in the widespread availability of PET-CT scanners [73]. Recent technological advances including ECG gating alongside improved PET resolution and fusion with detailed CT angiography of the coronary vessels have allowed translation of this technology into the heart. Theoretically any pathological process can be studied dependent on a suitable radiotracer being developed so that in principle any of the above characteristics of high-risk plaque can be targeted. However, to date the most promising studies investigating coronary atherosclerosis have utilised the tracers18F-fluorodeoxyglucose (18F-FDG) as a marker of inflammation and 18F-fluoride as a marker of microcalcification.
8.3.7.1 Targeting Inflammation with 18F-Fluorodeoxyglucose
18F-fluorine-labelled 2-deoxy-2-fluoro-D-glucose (18F-FDG) is used extensively to image metabolically active cells with PET-CT. Indeed, it has been employed very successfully to identify metastases in patients with high-grade poorly differentiated tumours [73]. In cardiovascular disease, several studies have demonstrated a close association between 18F-FDG and tissue macrophage burden in the carotid arteries [74, 75]. Furthermore, 18F-FDG uptake is associated with traditional cardiovascular risk factors including age, male gender and metabolic syndrome as well as inflammatory biomarkers [76, 77]. Moreover, vascular 18F-FDG uptake is elevated in patients infected with human immunodeficiency virus [78] and with rheumatoid arthritis [79], perhaps accounting for the increased cardiovascular events in these patient populations. Indeed, 18F-FDG has become a widely used measure of vascular inflammation and employed to demonstrate the anti-inflammatory effects of statins and other lipid-lowering agents [80, 81]. However, recent data have suggested that the mechanisms underlying vascular 18F-FDG activity may be more complex, implicating hypoxia as an important driver of 18F-FDG uptake by both macrophages and vascular smooth muscle cells [82]. Recent data have also suggested that 18F-FDG preferentially labels M1-type pro-inflammatory macrophages rather than the anti-inflammatory M2 type [83].
Whilst 18F-FDG uptake has been validated in large vessels such as the aorta, carotid, iliac and femoral arteries, attempts to utilise this tracer in the coronary arteries have been less successful. Increased 18F-fluorodeoxyglucose in the coronary arteries has been described in patients with coexisting malignancy [84, 85]. Since then, three prospective studies have examined the feasibility and reproducibility of assessing uptake of this tracer in the coronary vasculature [86–88]. Whilst two early studies [86, 88] suggested that 18F-fluorodeoxyglucose might identify some inflamed plaques in patients with recent myocardial infarction, others have more recently demonstrated that in 50 % of patients with acute myocardial infarction, there was no uptake of 18F-fluorodeoxyglucose in the culprit plaque [88].
We reported the coronary uptake of 18F-FDG in patients with and without aortic stenosis (Fig. 8.2) [87]. The 18F-FDG uptake was difficult to quantify, particularly in the left main stem and circumflex artery. It was not possible to quantify accurately in 49 % of the vessel territories examined in this cohort. This was largely the result of myocardial spillover into the coronary arteries, which was observed despite the dietary restrictions imposed in the study. Overall, we did not find any difference in patients within control group and patients with atherosclerosis.
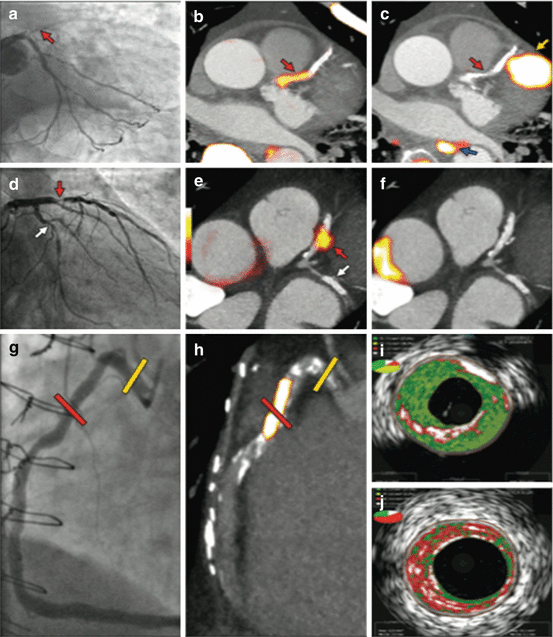
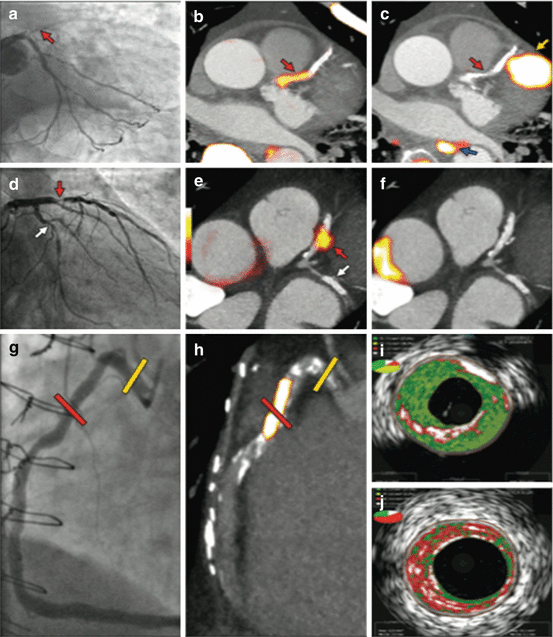
Fig. 8.2
18F-fluoride and 18F-FDG uptake in patients with myocardial infarction and stable angina: Focal 18F-fluoride and 18F-fluorodeoxyglucose uptake in patients with myocardial infarction and stable angina. Patient with acute ST segment elevation myocardial infarction with (a) proximal occlusion (red arrow) of the left anterior descending artery on invasive coronary angiography and (b) intense focal 18F-fluoride uptake (yellow-red) at the site of the culprit plaque (red arrow) on the combined positron emission and computed tomogram (PET-CT). Corresponding 18F-fluorodeoxyglucose PET-CT image (c) showing no uptake at the site of the culprit plaque (18F-FDG). Note the significant myocardial uptake overlapping with the coronary artery (yellow arrow) and uptake within the oesophagus (blue arrow). Patient with anterior non-ST segment elevation myocardial infarction with (d) culprit (red arrow; left anterior descending artery) and bystander non-culprit (white arrow; circumflex artery) lesions on invasive coronary angiography that were both stented during the index admission. Only the culprit lesion had increased 18F-NaF uptake on PET-CT (e) after percutaneous coronary intervention. Corresponding 18F-fluorodeoxyglucose PET-CT showing no uptake either at the culprit or the bystander stented lesion. Note intense uptake within the ascending aorta. In a patient with stable angina with previous coronary artery bypass grafting, invasive coronary angiography (g) showed non-obstructive disease in the right coronary artery. Corresponding PET-CT scan (h) showed a region of increased 18F-NaF activity (positive lesion, red line) in the mid-right coronary artery and a region without increased uptake in the proximal vessel (negative lesion, yellow line). Radiofrequency intravascular ultrasound shows that the 18F-NaF-negative plaque (i) is principally composed of fibrous and fibrofatty tissue (green) with confluent calcium (white with acoustic shadow) but little evidence of necrosis. On the contrary, the 18F-NaF-positive plaque (j) shows high-risk features such as a large necrotic core (red) and microcalcification (white) (Reprinted from Joshi et al. [89] under CC BY open access)
More recently, we have prospectively evaluated 18F-FDG in patients with stable and unstable coronary disease [89]. Again, we failed to demonstrate the utility of this tracer in identifying culprit or high-risk plaques in patients with myocardial infarction or stable angina, primarily due to intense myocardial uptake of this tracer that hampered assessment in the coronary arteries. This was despite stringent dietary recommendations that successfully suppressed myocardial uptake in 70–85 % of patients, a rate that compares favourably with previous studies (57–84 %) [86, 88]. However, frequently this suppression resulted in a patchy distribution of myocardial uptake that obscured activity in one or more coronary vessels. Given its limitations, we believe that 18F-FDG is unlikely to become sufficiently robust to permit its clinical application to the coronary circulation unless we find a more effective way to suppress myocardial uptake. However, we believe that 18F-FDG uptake remains a very important measure of general vascular inflammation and will remain the principal tracer in assessment of inflammation in large arteries such as the aorta and carotid arteries.
Whilst advances in suppressing myocardial 18F-FDG activity and cardiac motion correction are likely to improve this technique, ultimately we believe that more macrophage-specific tracers will be required. Indeed, the development of such tracers is an active area of research with several agents, including 1C-PK11195 [90] and 18F-fluorodeoxymannose [3], holding real promise. Specifically, 18F-fluorodeoxymannose (FDM) also binds to mannose receptors present on activated anti-inflammatory (M2) macrophages that are associated with plaque features of high risk such as neovascularisation and intra-plaque haemorrhage. By targeting mannose receptor expression in addition to plaque glucose metabolism, 18F-FDM is potentially able to both quantify and characterise plaque inflammation.
8.3.7.2 Targeting Plaque Necrosis and Microcalcification with 18F-Fluoride
18F-fluoride is a PET tracer with favourable pharmacokinetic properties, first introduced by Blau and coworkers in 1962 for study of bone diseases [91–96]. 18F-fluoride uptake is characterised by excellent tissue to background ratios allowing an accurate assessment of tracer activity within the vascular tissue. Specifically, the tracer exchanges with hydroxyl groups in regions of hydroxyapatite at the surface of the bone to form fluorapatite [91–93]. In so doing, 18F-fluoride acts as a marker of bone mineralisation and has been extensively utilised to study various bone-related clinical conditions [14, 97–103], including the detection of malignant bone involvement [104–108].
The mechanisms of 18F-fluoride uptake within the vasculature are similar to those observed in the bone. 18F-fluoride primarily highlights areas of vasculature undergoing active calcification. The degree of 18F-NaF uptake is dependent on the surface area of the hydroxyapatite crystalline structure [109]. As a consequence of this surface area effect, 18F-NaF preferentially binds to regions of this newly developing calcification as opposed to field macroscopic calcification where much of the hydroxyapatite is internalised and not available for binding. Within an atherosclerotic plaque, calcification is believed to occur as a direct response to intense necrotic inflammation similar to that observed in caseating granulomata. Hydroxyapatite crystals are laid out within the areas of necrosis to which 18F-fluoride binds. Indeed, recent unpublished data suggests that 18F-fluoride is a specific marker of calcification activity within atherosclerotic tissue [110].
The vascular uptake of 18F-fluoride has been recently described in large vessels such as the aorta, carotids and femoral vessels. In the latter 18F-fluoride uptake has been shown to correlate with cardiovascular risk factors and plaque burden [111–113]. However, only a fifth of all calcified plaques on CT demonstrated increased 18F-fluoride uptake, highlighting that this tracer provides different information to CT [114]. Furthermore, the distribution of 18F-fluoride and 18F-FDG uptake demonstrated poor co-localisation confirming that these two tracers highlight distinct pathological processes [115].
Coronary 18F-fluoride has been subsequently described in the patients with and without aortic stenosis with excellent reproducibility (Fig. 8.2) [87, 116]. Furthermore, the ability of this tracer to distinguished patients with increased cardiovascular risk scores, prior MACE and patients with higher rates of angina symptoms has been demonstrated [87]. 18F-NaF uptake in the coronary arteries demonstrated a different distribution to the presence of macroscopic calcium detected by computed tomography. Indeed, we observed that 41 % of patients with very high coronary artery calcium scores (above 1000 Agatston units) did not have increased 18F-NaF uptake, suggesting the ability of 18F-fluoride to distinguish between patients with dormant pacified calcific disease and metabolically active ongoing calcification.
Subsequently, 18F-fluoride has been studied in patients with myocardial infarction, stable angina and symptomatic carotid disease [89]. Amongst 40 patients with ST and non-ST elevation myocardial infarction, increased 18F-fluoride uptake was observed at the site of the culprit plaque in 93 % of patients. Indeed, activity was around 30 % higher in the culprit plaque than the maximum activity recorded anywhere else in the coronary vasculature, independent of the effects of coronary artery stenting. In patients with symptomatic carotid disease who had undergone carotid endarterectomy, 18F-fluoride signal co-localised to the site of macroscopic plaque rupture. Histological analysis of these 18F-fluoride-positive regions of atheroma showed increased levels of macrophage infiltration, cell death, necrosis and calcification activity as compared to 18F-fluoride negative areas.
In patients with stable angina, about two-fifths of patients with stable angina had increased fluoride uptake with 18F-fluoride-positive plaques demonstrating multiple features of plaque vulnerability including a higher necrotic core burden, positive remodelling and microcalcification as compared to plaques without uptake.
What then is the explanation for 18F-fluoride identifying ruptured and high-risk coronary atheroma? As in bone, 18F-fluoride is believed to bind to hydroxyapatite proportional to the available surface area of this crystal for binding. This is much higher in regions of nanocrystalline microcalcification than the regions of macroscopic calcium traditionally identified by CT. Indeed, this has been confirmed in recent mechanistic studies and explains the different information provided by these two imaging modalities and the ability of 18F-fluoride PET to identify high-risk lesions with newly developing microcalcification, the latter presumably occurring as a response to intense inflammation within the necrotic core of these plaques.
The next question is whether all plaques with increased 18F-fluoride activity will go on to rupture and cause myocardial infarction. We believe this is highly unlikely. In the first instance, the calcific healing response to intense necrotic inflammation is often likely to prove successful in pacifying the plaque and avoiding rupture. Moreover, we know that even if the plaque does go on to rupture, this is actually a ubiquitous event that most commonly is subclinical and does not result in myocardial infarction. Nonetheless, we believe that 18F-fluoride will have a role in identifying the vulnerable patient, those subjects with high-risk atheroma that will be at an elevated risk of an event compared to subjects with truly stable coronary artery disease [117]. This issue will be addressed in the ongoing multicentre prospective study PREFFIR which will investigate whether 18F-fluoride PET imaging can prospectively predict plaque rupture events and disease progression in patients with coronary artery disease. The DIAMOND study will then go on to investigate whether such patients might then benefit from aggressive medical therapy.
8.3.7.3 Alternative Novel Radiotracers
The great strength of PET imaging is that tracers can ultimately be developed to target any pathological process of interest and can therefore be directed to any of the features that characterise high-risk plaques. Whilst this is an expensive process, the studies using 18F-fluoride have clearly demonstrated that metabolic plaque imaging in the coronary arteries is now feasible, making an expansion of activity in this area likely.
Given its pathophysiological importance, inflammation would appear to be the prime potential target. As discussed, 18F-FDG, whilst an excellent tracer for measuring inflammation in the aorta and carotids arteries, is of limited use in the coronary arteries, the metabolic activity of which governs the majority of clinical events. There is therefore an urgent need to develop more macrophage-specific tracers that crucially do not demonstrate increased activity in adjacent structures as the myocardium.
Inflammation Imaging with 68Ga-DOTATATE
There has been significant interest in inflammation imaging with 68Ga-DOTATATE, a novel tracer with receptor binding specificity to somatostatin 2. Although primarily overexpressed in malignancies, in particular neuroendocrine tumours, somatostatin receptors are thought to be preferentially expressed in damaged and proliferating endothelium and by activated macrophages [118–120]. Indeed, murine data has shown good correlation between tracer activity and macrophage density in atherosclerotic lesions [121]. Observational data from oncology patients has reported uptake for this tracer in large vessels again demonstrating a good correlation with calcific plaque burden. Interestingly, in this study, 18F-FDG uptake did not co-localise with 68Ga-DOTATATE suggesting labelling of distinct process within vascular inflammation [122]. Furthermore, uptake has been described in the coronaries with modest correlation with calcific plaque burden and previous cardiovascular events [123, 124].
Inflammation Imaging with 11C-PK11195, 18F-FMCH and 11C-Choline
11C-PK11195 is a selective ligand for peripheral benzodiazepine receptors expressed in activated macrophages and is primarily used to image neuro-inflammation. It has been recently investigated in atherosclerotic plaque imaging. In preclinical models, 11C-PK11195 localised to areas of vascular inflammation [125] and has also been investigated for the use in atherosclerosis imaging. Indeed, carotid uptake of 11C-PK11195 has been shown to be increased in symptomatic carotid atherosclerosis, with good correlation between the tracer autoradiography and CD68 macrophage expression in ex vivo plaques [126]. Uptake of 11C-PK11195 has also been described in patients with active systemic vasculitis [127].
Other tracers that are thought to bind to activated macrophages include 18F-FMCH and 11C-choline. Primarily used in cancer imaging, the tracers are thought to be incorporated within the cell membrane after phosphorylation by choline kinase [128]. Similar to other tracers, uptake has been described in murine models of atherosclerosis and oncological patients in retrospective analysis [129–132].
8.3.8 Assessment of Hypoxia with 18F-Fluoromisonadazole (FMISO) and Neoangiogenesis with 18F-Galacto and 18F-Fluciclatide
Other important features of plaque vulnerability apart from inflammation include hypoxia and neoangiogenesis, both of which are legitimate atherosclerotic targets. Tissue hypoxia within the plaque increases with the size of the necrotic core and creates a stimulus for new microvessel formation through hypoxia-inducible factor 1α [133]. Newly formed microvessels are fragile and permeable without lack of supporting structures and are prone to intra-plaque haemorrhage [37, 134]. Extravasation of erythrocytes within the plaque is a rich source of free cholesterol and cholesterol ester, which play an important role in plaque progression and vulnerability.
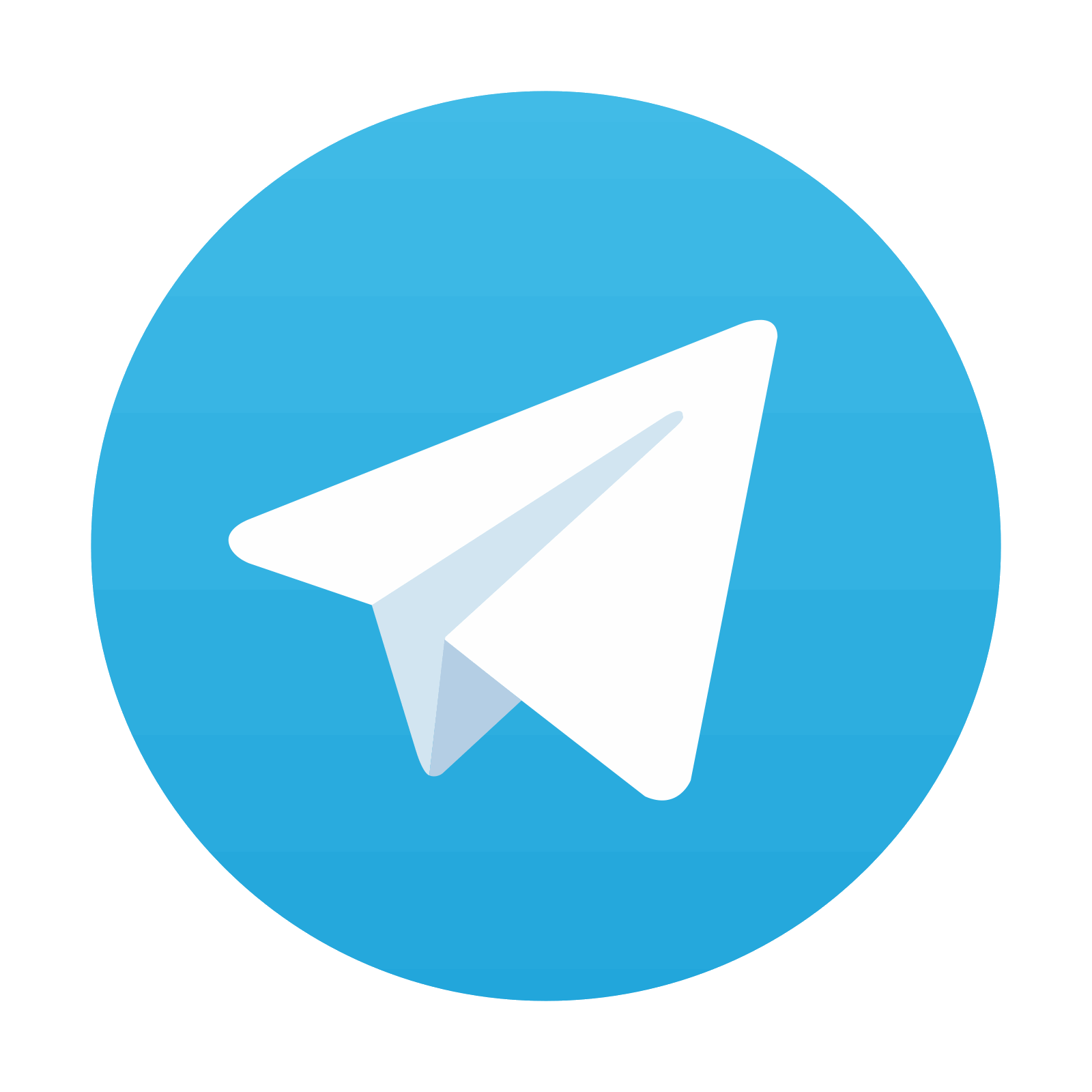
Stay updated, free articles. Join our Telegram channel
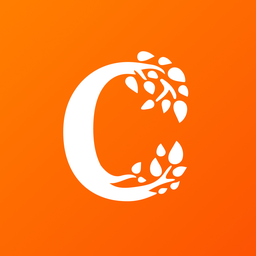
Full access? Get Clinical Tree
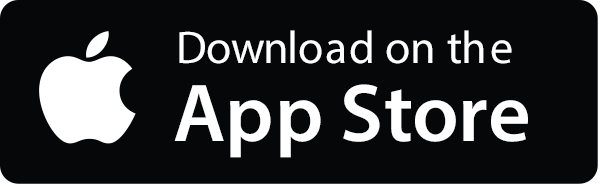
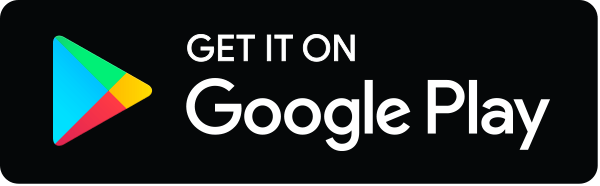