6 Magnetic resonance imaging (MRI) is an exceptionally powerful and safe imaging modality. In contrast to many of its radiologic counterparts, the modality does not expose the patient or the operator to ionizing radiation and therefore avoids the principle safety concern in x-ray-based imaging systems. The MR environment, however, is quite complex and there are aspects that pose substantial, but largely avoidable, safety hazards. Most notably, MRI systems are always “on” in the sense that safety hazards exist even when the system is inactive. This is in contrast to virtually all other radiologic systems and represents a substantial educational challenge for patients and personnel who work in and around MRI suites. In this chapter, we address the many facets of MRI safety and pay particular attention to the implications for interventional procedures. Many of the safety concerns related to MRI are heightened in the interventional setting as a result of the additional equipment and personnel that enter the MR environment. Thus particular attention needs to be paid to suite layout and access, personnel training and screening, and equipment safety testing and labeling. The fundamental basis for safety concerns in MRI will initially be reviewed and then followed by a summary of safety protocols that are commonly employed to minimize risk in diagnostic MRI settings. Impending occupational exposure limits for the various magnetic fields that are created in MRI systems will also be introduced and evaluated for the potential impact on interventional MRI approaches. Finally, recommendations for safety protocols in interventional MR systems will be addressed. MR systems are composed of three major subcomponents: (1) the static magnetic field (B0), (2) the time-dependent gradient fields (dB/dt), and (3) the radiofrequency (RF) transmission system (B1). Each of these components produce distinct safety considerations and thus will be considered separately. The most widely recognized safety concern with MR imaging relates to the presence of a large, invisible, static magnetic field. The static magnetic field of commercially available MR systems is created with either superconducting or permanent magnets. The primary objective of these designs is to create a very strong and homogeneous magnetic field over a modestly sized volume. Secondary criteria, which have become increasingly important, are to maximize system openness and minimize peripheral magnetic fields. The clinical standard for whole-body MRI systems is well established at 1.5 or 3.0 tesla (T), which is achieved with cylindrical bore superconducting magnets. Lower field strength systems featuring enhanced lateral access are also commercially available for clinical use. Higher field strength magnets for research purposes are becoming more prevalent, but are unlikely to be considered for interventional applications. The static magnetic field has several different regions, ranging from magnet isocenter, where there is a very high and homogeneous magnetic field over a relatively small volume, to the magnetic fringe field, where relatively low magnetic fields extend over large areas. An important transitional zone occurs as you near the magnet, as the magnetic field increases rapidly, producing the potential for magnetic attraction of ferrous objects. The area surrounding the physical magnet produces the potential for substantial deflection forces and torques in magnetic objects. The magnitude of deflection forces is directly proportional to the spatial rate of change in magnetic field and the mass and magnetization of the magnetic object. Thus heavier magnetic objects such as ferrous gas cylinders will experience proportionately greater force than objects with less mass or less inherent magnetization. The spatial rate of change in magnetic field is typically greatest near the magnet aperture, where the field strength is rapidly rising toward its eventual peak value. The spatial rate of change is actually enhanced on shielded magnets, which are designed to minimize magnetic fields outside of the magnet bore. Accordingly, magnetic fields are dampened in the periphery of these magnets, but the magnetic attraction near the bore is enhanced. Furthermore, the rate of magnetic field change increases rapidly as you approach the magnet. This creates a circumstance whereby the magnetic force substantially increases as magnetic objects move even slightly closer to the magnet bore and makes it very difficult to hold back an object once it has begun to be attracted toward the magnet. Unfortunately, projectiles are not uncommon in MRI suites. In the vast majority of cases these projectiles are small objects that are pulled from patients or staff as they approach the magnet. However, larger objects, including gas cylinders,1 floor waxing machines, and chairs, have been reported, which have resulted in serious injury and death. Protection from these types of accidents requires strict institutional policies and personnel adherence to the established safety and screening protocols. The large static magnetic field of MRI systems poses a very significant safety hazard to patients with internalized magnetic objects. Metallic implants and foreign bodies will experience the same forces described above and may move if not well seated in tissue. There is particular concern for metallic objects in the eye, which can produce ocular injury when exposed to high magnetic fields.2 There is further concern for implanted medical devices such as aneurysm clips,3 which experience deflection and force that may have devastating effects. In general, aneurysm clips made from ferromagnetic materials are contraindicated for MRI, whereas weakly or nonferrous clips are considered safe. Other endovascular devices, including stents, coils, and filters, tend not to be made of strongly ferromagnetic magnetic material and are permitted in MRI scanners. However, it is recommended to wait several weeks after placement of endovascular devices made from weakly ferromagnetic materials to allow for endothelialization and more secure seating of the device. There are many other medical implants that may experience forces when exposed to the static magnetic field of an MRI scanner. An excellent and commonly used reference for determining the safety status of a wide range of implants and devices is maintained by Dr. Frank Shellock and is available online (mrisafety.com) and in book form.4 The majority of MRI systems presently operate with a superconducting magnet producing the static magnetic field. These systems require that the conductive windings that create the static magnetic field be cooled to very low temperatures, which is typically achieved by immersing them in liquid helium. Superconductive windings offer no resistance, so once the appropriate current has been injected into the structure it will maintain at that current level, and corresponding magnetic field, indefinitely. Thus this component of the MR system is “always on” and must always be considered. Superconductive systems, however, also pose another safety risk related to the presence of the cryogenic system. If for some reason the superconductor develops resistance, its temperature can rise very quickly due to the high current levels that run in its windings. This phenomenon is referred to as a “quench” and results in rapid loss of the magnetic field and energy transfer from the coil windings to the cryogenic liquid. The liquid helium is elevated above its boiling point, creating a much larger volume of helium gas that must escape the cryostat. MR systems are designed with vents to permit safe expulsion of helium gas, but if this system fails then the gas will rapidly displace air in the magnet room. The loss of oxygen in the magnet room, coupled with the increased pressure as a result of the rapid expulsion of helium gas, can have tragic consequences. Thus the magnet room should be equipped with outward opening doors and be rapidly evacuated in the event of a magnet quench. Humans are continuously exposed to a low-level relatively static magnetic field produced by the molten iron core of the earth. This ubiquitous magnetic field varies between 0.3 to 0.6 gauss over the surface of the earth and poses little concern. Higher, time-varying, magnetic fields are associated with man-made creations such as power lines and substantial study has been made of the potential biologic impact of exposure to these fields. There has been particular concern about a possible correlation between exposure to these electromagnetic fields and cancer, although a causal relationship between the two has never been definitively established.5 The static magnetic field of an MR imager, however, is quite different. It is static, much like the earth’s magnetic field, but has substantially higher field strength. Although human exposure to the magnetic field strengths associated with MR systems is relatively new, there has been substantial study on the biologic effects of these fields. Schenck provides a comprehensive review6 of the studies that have been done and the associated findings. The impact of static magnetic fields on everything from gene expression to circadian rhythms has been evaluated without demonstration of a clearly pathologic effect from the presence of static magnetic fields of the magnitude used for clinical MRI. However, this remains an important area of study and assumptions about the potential for, or lack of, biologic effects from all forms of magnetic fields should not be made until carefully controlled studies have been performed. Spatial localization in MR imaging is achieved via the transient application of magnetic field disturbances. The strength of these magnetic fields is substantially less than the static magnetic field, but they are rapidly activated and deactivated during MR scanning. dB/dt is the ratio between the amount of change in amplitude of the magnetic field (dB) and the time it takes to make that change (dt). This temporal variation in magnetic field induces electric fields and forces that create an assortment of other potential safety concerns. The electric field that is created when imaging gradients are activated or deactivated has the potential to produce nerve stimulation. The potential for this stimulation is dependent on both the strength and duration of the induced electric field.7 Because the strength of the magnetic field produced by gradient coils increases with the distance from the isocenter, peripheral nerve stimulation (PNS) is most commonly experienced. Patients can experience a tingling sensation or twitching once a threshold level has been exceeded. This can progress to become painful with relatively modest increases beyond the stimulation threshold.8,9 At sufficiently high levels, nerve stimulation could potentially induce epileptic seizures or produce cardiac stimulation. These side effects range from minor discomfort to potentially catastrophic; they place a practical biologic limit on the performance of imaging gradients. Gradient coil design also plays a role in determining if and where nerve stimulation may occur,10 and designs for high performance gradients that minimize the level of stimulation generating electric fields are being explored.11 MR systems emit noise while scanning due to the activation and deactivation of the gradient coil system. Linear perturbations of the static magnetic field are transiently created by running current through gradient coil windings that are built into the scanner bore. Substantial forces in the gradient coil windings are induced when current levels change, making the wires want to twitch. The coil windings cannot move because they are embedded in a solid former, but these forces produce a small vibration in the structure. Because gradient switching occurs in the same frequency range (~ kHz) as human hearing, the vibration is emitted as an audible noise. The amplitude and frequency of the emitted noise is both a function of the MR system and the specific pulse sequence that is being performed, but can be appreciable. The acoustic noise emitted by an MR system can produce temporary hearing loss or permanent impairment and therefore exposure must be limited. Patients within the magnet bore must wear hearing protection and anyone remaining in the magnet room during scanning must similarly consider ear protection.12 The necessity for ear protection is a function of the amplitude, frequency, and duration of the acoustic exposure and agencies such as the Occupational Safety and Health Administration (OSHA) in the United States set exposure limits. Fortunately, the level of acoustic noise produced by today’s MR systems are adequately mitigated by an assortment of hearing protection devices. MRI requires that the protons within the bore of the magnet be excited from their equilibrium state. The signals that are used to create MR images reflect the signal emitted by these protons as they subsequently return to their equilibrium state. System excitation is achieved by exposing the subject to energy at the resonant, or Larmor, frequency of protons in the static magnetic field. For commercial MR systems this resonant frequency lies in the radiofrequency (RF) portion of the electromagnetic spectrum (i.e., 64 MHz at 1.5 T). This energy is well below the ionization threshold; therefore, it will not directly damage molecules in the same way ionizing radiation can. The primary concern with RF energy is heating related to absorption of this energy.13
Safety Considerations
Safety Concerns of Magnetic Resonance Systems
Static Magnetic Field (B0)
Projectile Risk
Internal Ferrous Objects
Cryogenic Systems
Human Exposure to Magnetic Fields
Time Varying Gradient Fields
Nerve Stimulation
Acoustic Noise
Radiofrequency Transmission
Specific Absorption Rate
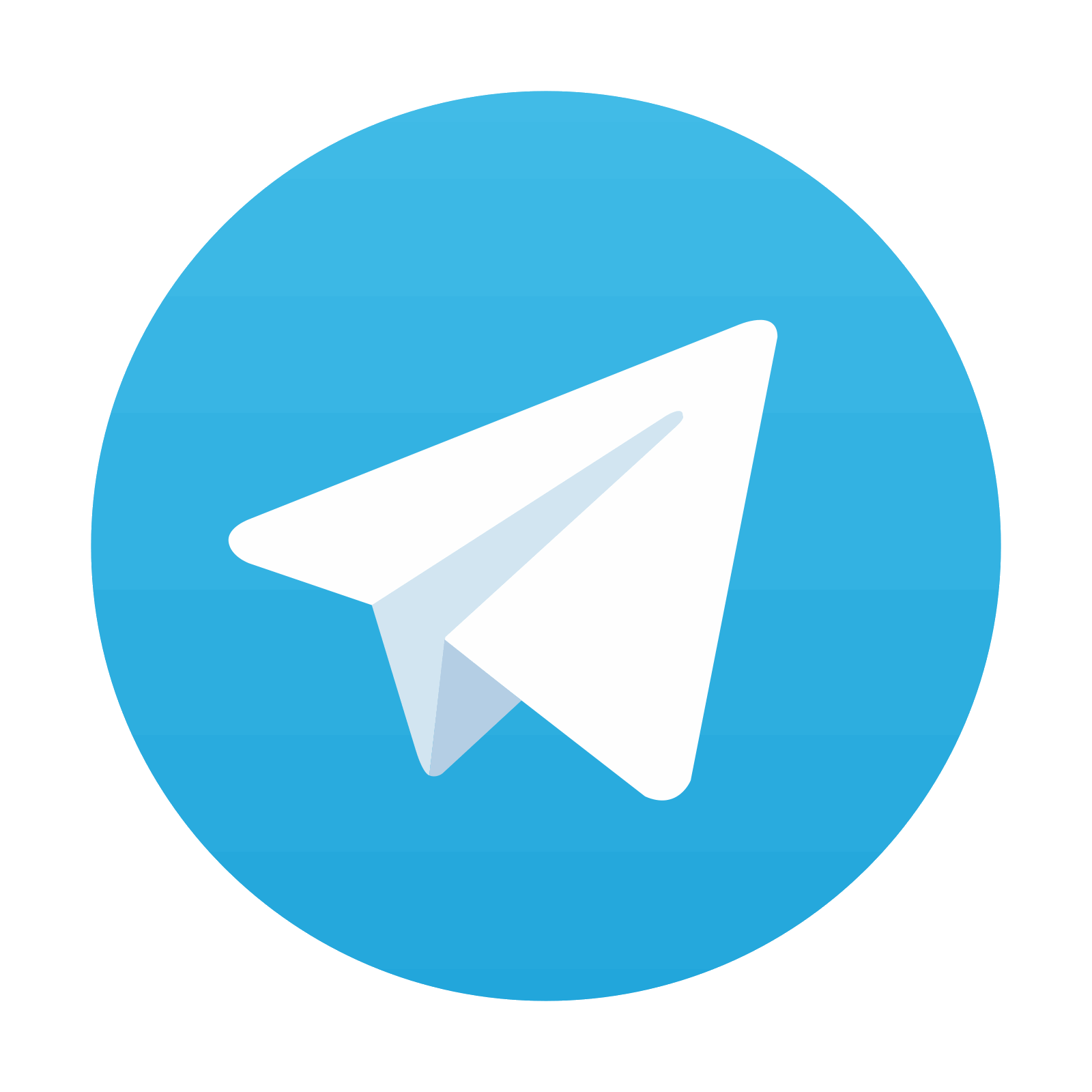
Stay updated, free articles. Join our Telegram channel
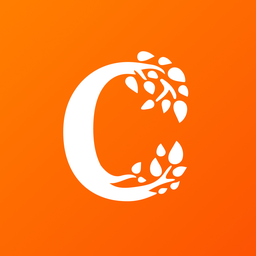
Full access? Get Clinical Tree
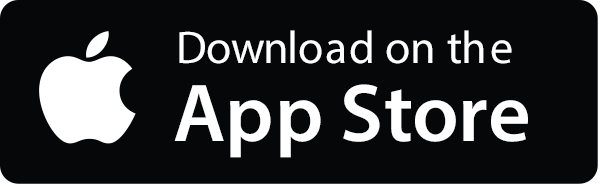
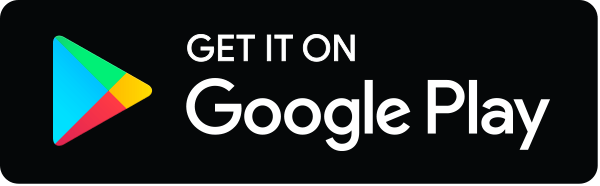