1.1 Technical requirements of a stereotactic radiosurgery system
Stereotactic radiosurgery (SRS) requires high doses of radiation to be delivered to a prespecified volume of tissue with remarkably high spatial accuracy within a reasonable treatment time. Typically, the accuracy of SRS dose delivery must be < 1 mm. The parallel evolution of imaging and radiation therapy technologies in the late 20th century was essential to the development of SRS. To achieve submillimeter accuracy in delivery, very high-resolution imaging modalities are essential to identify the anatomical target volume(s). Multiple imaging modalities, such as computed tomography (CT), magnetic resonance imaging (MRI), positron emission tomography (PET)/CT, three-dimensional rotational angiography (3D-RA), are fused to allow the neurosurgeon and/or the radiation oncologist to contour the 3D target(s) and normal tissue structures accurately on the fused datasets. Additionally, the coordinate system of the primary imaging dataset, typically CT scan images, must be perfectly matched to the coordinate system of the treatment machine. With highly accurate knowledge of the 3D target, the treatment machine must be able to deliver a spatial dose distribution that is accurate to < 1 mm.
A second requirement of a radiosurgery delivery system is that it can deliver an ablative dose to the entire target tissue while generating a sharp falloff of dose outside of the target volume. To reduce the potential toxicity associated with SRS, the normal tissue that abuts the target must be constrained to receive a nontoxic dose. This requires that the radiation beams used for SRS must be much smaller than those used for conventional radiotherapy. Furthermore, the 3D shape of some SRS targets (e.g., meningiomas, acoustic neuromas, arteriovenous malformations) is often highly irregular. The SRS delivery system must therefore have a mechanism allowing it to produce irregular dose distributions in three dimensions.
To achieve accurate dose delivery, knowledge of the location of the target volume and normal tissue volumes (i.e., avoidance structures) must be known with high precision. The locations of these structures must be known in the treatment coordinate system throughout the duration of treatment. No patient can hold his head absolutely still for minutes or hours; this means that either some mechanism to immobilize the head—or a mechanism that dynamically monitors and corrects for patient motion—must be incorporated into the SRS treatment system.
1.2 Radiation types used in SRS
Radiation is the transmission of energy as electromagnetic waves or as moving atomic or subatomic particles. Ionizing radiation in the electromagnetic spectrum extends from ultraviolet light through gamma rays and X-rays. In radiation oncology, it was recognized in the 20th century that ionizing radiations in the gamma and X-ray ranges can cause lethal and sublethal damage at the cellular level. By ejecting electrons from atoms within tissue, ions and free radicals are formed, which can cause double-strand and single-strand breaks in a cell’s DNA. Normal cells have a better ability to repair DNA damage than malignant cells. Furthermore, ionizing radiation can cause changes in the intracellular and extracellular microenvironment. There are many ongoing studies on the precise ways that high-dose radiation, delivered in a single (or up to 5) session(s), interacts with cells to cause cell death, including to stimulate apoptotic pathways, stimulate the immune system, compromise the blood supply to the tumor, etc.
Short wavelength electromagnetic radiations are mostly used for radiosurgery. X-rays and gamma rays are both classified as “photons” or discrete energy packets of electromagnetic energy. X-rays are photons that are generated by machines, while gamma rays are generated by radioactive decay. Currently, there are a limited number of proton SRS treatment centers in the United States and around the world. Heavy ion machines are currently in use for medical purposes in a small number of centers in Europe and Japan. Therefore, proton and heavy ion machines will not be covered in this chapter.
1.2.1 Radioactive materials systems
Nuclear reactors were developed during World War II as part of the Manhattan Project. After the war, Dr. Harold Johns (University of Saskatchewan) asked the Canadian national reactor to begin production of Cobalt-60 for medical applications. When nonradioactive Cobalt-59 is bombarded by neutrons, it becomes radioactive Cobalt-60 ( 60 Co). 60 Co decays via β decay to an excited state of Nickel-60, which then emits two high energy (1.17 MeV and 1.33 MeV) gamma rays. These energetic gamma rays can penetrate deeply into the body, making them useful in clinical radiation therapy. Furthermore, the half-life of 60 Co decay is 5.27 years, so the useful life of this source is acceptable for hospital use.
When photons interact with any material, they deposit their energy exponentially. A single beam of 60 Co radiation falls to about 50% of its entrance dose after passing through 10 cm of tissue. For a 10-cm deep tumor, the dose to the skin would then be about twice that of the tumor dose. Therefore, a single beam was found to cause unacceptable skin toxicity. Multiple beams from different angles in one plane around the patient, aiming at the same point at the center of the tumor, would reduce the dose to the skin while accumulating a tumoricidal dose at 10 cm. For this reason, Dr. Johns and his team mounted a 60 Co source on a gantry that rotated around the patient, either statically at multiple angles or in full or partial arcs. The first use of this “teletherapy” machine in the United States was in 1961, and thereafter, machines spread to cancer centers throughout the world during the 1960s and 70s.
1.2.1.1 Lars Leksell’s seminal insight
Using 60 Co, Swedish neurosurgeon Dr. Lars Leksell developed the first Gamma Knife unit in 1968 as part of his ongoing search for a noninvasive way to treat functional brain disorders. Dr. Leksell’s idea was that he could deliver a much less toxic dose to surrounding normal tissues if the radiation beams were delivered from many different directions all around the head, all converging at the same point. This provided great dosimetric advantages in the achievable 3D dose distributions and normal tissue sparing compared to radiation delivered by a machine constrained to rotate in one plane, like Dr. John’s machine. Leksell arranged 201 individual 60 Co sources in a hemisphere around the patient’s head. Each source could be collimated to 4, 8, 14, or 18 mm diameters by heavy metal collimators. The collimators focused their “shots” of radiation such that they all converged at the same point in space, “the isocenter.” The 1968 prototype was used at the Karolinska Institute for 12 years.
Continued refinements through 1975 resulted in the forerunner of today’s Gamma Knife. The first Gamma Knife patient treatment in the United States was in 1987 at the University of Pittsburgh Medical Center. Since then, the design of the machine has advanced steadily over the years.
Leksell’s system required the use of a “frame” screwed to the cranium containing embedded radioopaque markers. The frame was attached to the cranium before imaging and only removed after completion of treatment. The frame accomplished two purposes: it kept the patient’s head from moving during imaging and treatment, and it established a stereotactic 3D coordinate system that would be identical for imaging and treatment.
Prior to being moved into the irradiation vault, the patient’s head was moved mechanically so that the lesion would be at the isocenter of the machine when the patient was moved into the irradiation chamber. The original Gamma Knife treatments were completed in one stage because reattaching a head frame multiple times was not desirable. The system was very efficient at delivering spherical and ellipsoidal dose distributions. However, to produce an irregular distribution, the treatment would need to be suspended, and the patient withdrawn from the vault. The patient’s head would be moved using micrometers in the x, y, and z directons. If needed, a different arrangement of collimator plugs was introduced into the helmet. When the patient was moved back into the treatment vault, a different part of the target volume would be irradiated. This was done as many times as needed until the superpoisition (sum) of the individual dose distributions nearly matched the irregular shape of the target. This, however, was quite time-consuming.
While the base concept remains the same, the Gamma Knife underwent many improvements over the years. In 2006, the Perfexion was introduced. In this system, the frame attaches to the treatment couch, which is motor controlled. The collimation system (helmet) is built into the vault, and collimator size changes are motor controlled. It uses three collimator sizes, viz. 16, 8, and 4 mm in diameter. It contains 192 60 Co sources that are mounted on eight movable sectors. These changes inproved treatment time significantly and reduced the possibility of manual error.
Elekta introduced the Icon system in 2015. It allows the use of a reloadable face mask (as opposed to a rigid frame), enabling multifraction treatments (2–5 sessions). It incorporates an onboard, cone-beam CT for more accurate patient setup. To sense patient movement during treatment, the system monitors the configuration of lights attached to the frame and to the patient’s nose, and will plug the collimators (stop irradiation) if a preset tolerance is exceeded.
By 2016, over 1 million patients had been treated using a Gamma Knife system, and over 2500 peer-reviewed publications were in print. Other technologies used for SRS today stand firmly on the vast mount of knowledge (e.g., effective target doses for various clinical abnormalities and safe doses to normal tissue structures) gleaned from the Gamma Knife experience.
There is, however, a downside to the use of Gamma Knife systems—source replacement can be expensive and resource-intensive. In the United States, transport and replacement involves the Department of Homeland Security (DHS), local police, the Nuclear Regulatory Commission (NRC), and State coordination due to safety and terrorism concerns. The machine must be taken out of clinical service during source replacement and the necessary subsequent quality assurance and safety checks. Because all radioactive materials have a half-life, the treatment time for a given patient on day 1 increases to twice that time in 5.27 years (the half-life of 60 Co), and four times that treatment time in about 10.5 years. If sources are not replaced regularly, the treatment times become impractical for patients and physicians who must attend every treatment.
1.2.2 Gantry-based linear accelerator systems
Linear accelerators (LINACs) have been used in Radiation Oncology for over 50 years. LINACs generate high energy photons by first injecting electrons generated by an electron gun into an accelerating waveguide. Electrons then acquire high energy from radiofrequency waves through the acceleration process and can exit the waveguide with an energy of 6 to 24 MV–SRS systems, typically using 6 MV photons. After exiting the waveguide, high energy electrons hit a high atomic number target (e.g., gold). Upon striking the target, electrons undergo an interaction called bremsstrahlung, producing a continuous spectrum of photons. The beam of high energy photons is collimated to the desired size before exiting the machine and traveling towards the patient.
Some gantry-based LINACs used for SRS are modified multipurpose LINACs with special software and hardware. The gantry (like a C-arm) and couch (turntable) each rotate in circular planes. The gantry and couch are aligned such that the points of intersection, as well as all beams, regardless of gantry and couch angle, coincide at the same point in space (isocenter). A secondary collimator is attached to the beam exit, further collimating the beam to discrete small diameters (e.g., 4–20 mm). Practitioners use either rigidly attached frames, like Leksell’s, or a noninvasively attached frame (“frameless” approach). Multipurpose LINACs were used for SRS in the 1980s and 90s. They generally have less spatial accuracy in dose delivery than systems specifically designed for SRS.
Nearly all gantry-based LINAC systems use gantry arcs in SRS treatment; the photon beam is on a predetermined arc during gantry rotation. The couch is then rotated to a different angle and another gantry arc is irradiated. The computerized treatment plan specifies the gantry angles and couch angles when the beam is on, the design of which permits hitting the target while avoiding passage of rays through radiosensitive normal structures. Originally, a single size collimator would be used for a given arc and then changed as specified in the treatment plan. Similar to the Gamma Knife approach, 3D shaped dose distributions were created by moving the patient relative to the machine. In other words, the isocenter was located at a different point in the lesion, and treatment was reinitiated.
In more recent years, manufacturers have added onboard imaging to the medical LINAC systems in order to assure the accuracy of patient setup. Some systems (e.g., Novalis) rely on stereotactic planar X-rays, where the X-ray tubes and image detectors are independent of the LINAC gantry and couch. The X-ray tubes operate in the kilovoltage range, producing images of diagnostic X-ray quality. Others use onboard X-ray tubes and detectors providing images at static gantry angles. Finally, another approach uses on-board cone beam CT (CBCT). In some systems, CBCT scans can be produced by kilovoltage X-ray tubes, or the MV treatment X-ray beam can also be used to produce images. Often, these imaging systems are used only prior to treatment, and therefore cannot detect or correct for patient motion during treatment. In later systems, intrafraction imaging can be obtained, where the treatment is stopped and the couch is automatically moved if a detected patient motion exceeds a preset tolerance level during treatment.
1.2.3 Non-gantry-based SRS systems
1.2.3.1 CyberKnife
After completing a fellowship with Lars Leksell using the Gamma Knife in Sweden, Dr. John Adler, a neurosurgeon at Stanford University, designed the novel noninvasive, frameless CyberKnife. The first system was installed at Stanford University in 1991. It was cleared by the Food and Drug Administration (FDA) for clinical investigation in 1994. Ultimately, the FDA cleared the system for the treatment of intracranial tumors in 1999 and tumors anywhere in the body in 2001. Today, over 1 million patients have been treated by a CyberKnife around the world, and there are many publications on its clinical efficacy and safety.
When it was introduced, the CyberKnife was unique in many ways. It uses a compact LINAC (X-band rather than S-band LINAC used in conventional radiation therapy) attached to a robot with 6 joints. The motion of the LINAC is not constrained to rotate in circles (arcs) around the patient. The robot moves the LINAC around the patient, such that 6 MV radiation beams can be aimed at the target lesion from thousands of angles. However, the beams are not constrained to hit the same point in the room (isocenter) like the Gamma Knife and gantry-based SRS systems. The robot can aim the beams at any point inside the target and multiple targets can be treated in the same session. Being nonisocentric, highly irregular dose distributions can be delivered in a reasonable treatment time. Typically, an optimized treatment plan includes delivering 100 to 300 beams per session.
Another unique attribute of the CyberKnife is that it can detect and correct for patient motion during treatment without the use of a frame attached to the cranium. The CyberKnife suite has an imaging system composed of ceiling and floor mounted X-ray tubes and detectors, producing pairs of stereoscsopic planar images before and during treatment. From the image pairs, the system can track the 6D location of the cranium (x, y, and z translations, as well as roll, pitch, and yaw angulations). Prior to treatment, this information is used to move the couch so that the cranium matches its position in the image datasets used for treatment planning. This establishes a common stereotactic coordinate system between the treatment plan and the treatment itself. It then takes new image pairs at preset time intervals during treatment and detects any cranial motion. It feeds that knowledge back to the robot, which then corrects the aim of the next X-ray beam to correct for any motion. The patient and the treatment table (couch) are not moved during teatment, rather the angle of the X-ray beam is changed to account for any motion of the cranium. This is called “skull tracking,” which relies on the invariance of the position of intracranial structures relative to the skull (rigid body geometry).
Collimation on the CyberKnife is accomplished in one of three ways. There are 12 fixed circular collimators with diameters between 5 and 50 mm. Any or all of them can be used for treatment, but each collimator change requires the robot to make one walk around the patient for each collimator size, which is time-consuming. Secondly, there is an automated, motor-driven collimator (called an iris, due to the similarity of the iris of the eye) that can change size at every beam angle, allowing optimized treatment in a shorter time and enabling precise matching of the dose distribution to the target shape. Finally, the newest systems incorporate a multileaf collimator (MLC), which also allows the beam shape to be noncircular and to change at every beam angle. This leads to even shorter treatment times, as well as excellent normal-tissue sparing with good target coverage.
1.2.3.2 Zap-X (2019)
Like the CyberKnife, the Zap-X system was the brainchild of Dr. John Adler. It is designed for intracranial and head and neck SRS therapy. It consists of a 3-MV X-ray LINAC and kV (diagnostic) imaging system mounted on a fixed structure that moves around the patient’s head in a gyroscopic manner. Similar to the CyberKnife, the imaging system detects and corrects for patient motion during treatment. The patient is inside a shielding capsule during treatment, which virtually eliminates the need for room shielding. According to Dr. Adler, the objective of designing this novel system was to provide a system that would be affordable worldwide. The capsule shielding is sufficient to allow the staff to be in the room with the patient during treatment. This can reduce patient anxiety as well as the space allocated by the facility for SRS. The reduction in the need for room shielding also reduces the construction costs of the unit. The system received regulatory approval in 2017, and the first patient was treated using this system at Barrow Neurological Institute in 2019. Since then, Zap-X systems have been installed in various countries around the world.
1.3 Future directions
MRI-guided LINACs (MRL) received FDA approval in 2018. The clinical implementation of 1.5 Tesla MRL (e.g., Elekta Unity) and 0.35 Tesla MRL (ViewRay MRIdian) for SRS and streotactic body radiation therapy (SBRT) is currently in progress.
References
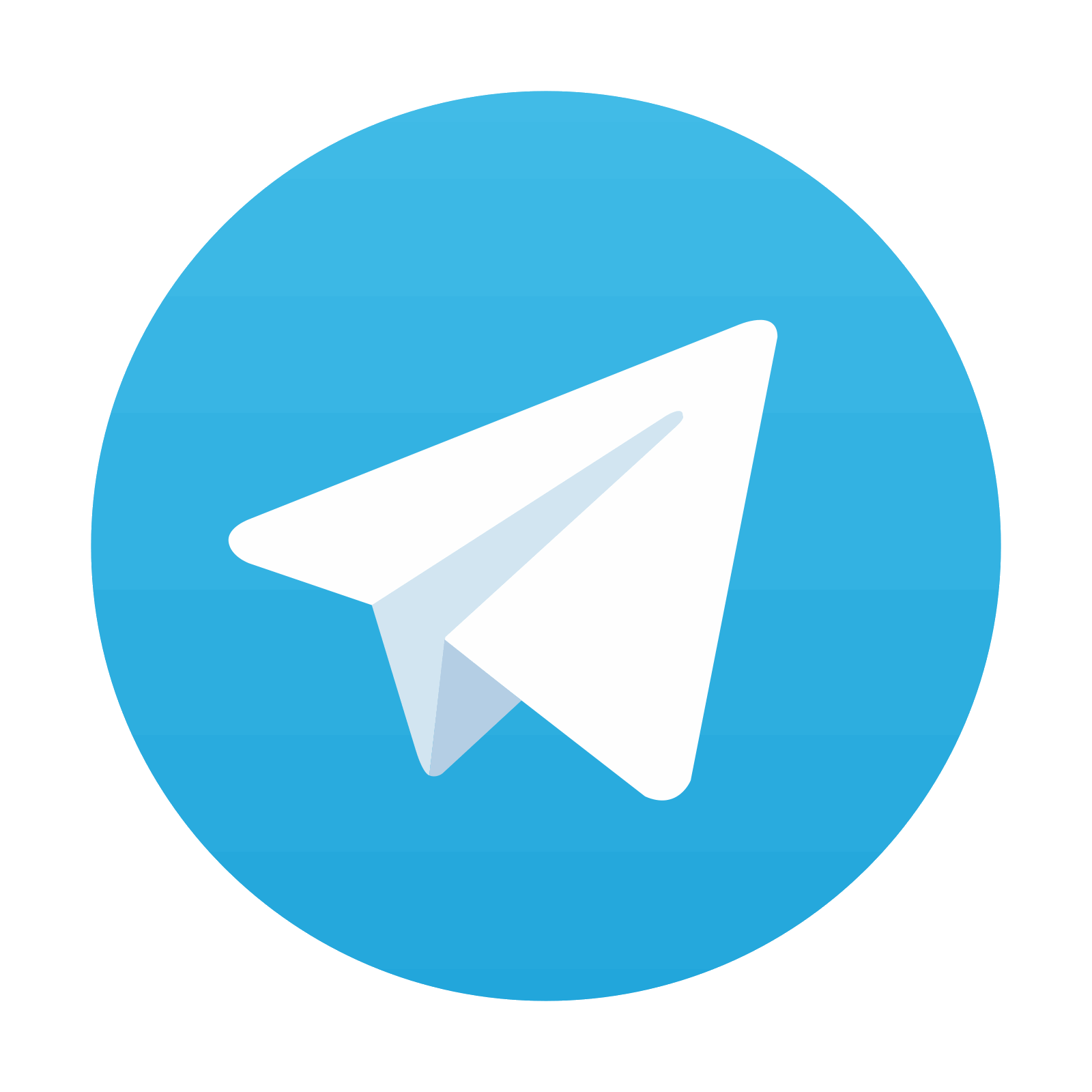
Stay updated, free articles. Join our Telegram channel
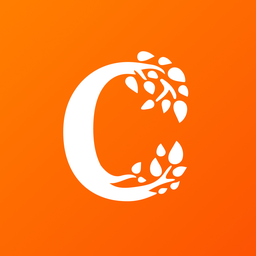
Full access? Get Clinical Tree
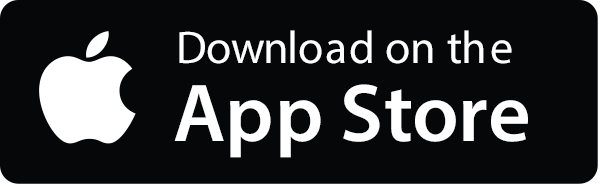
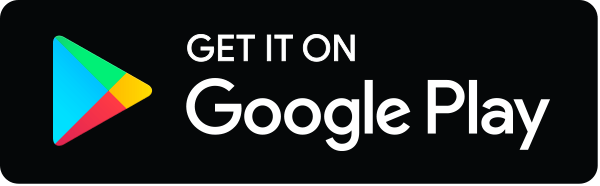
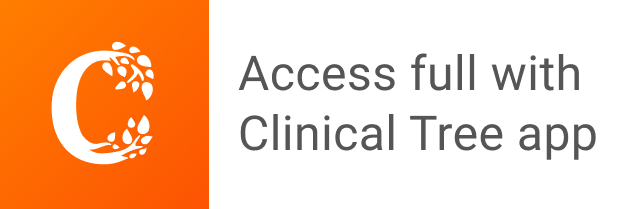