Chapter 38 Ultrasound
Introduction
Since the introduction of ultrasound to medical imaging in the 1960s its popularity has grown and applications widened into numerous subspecialties of medicine. Excluding plain radiography, ultrasound scans are the most commonly undertaken diagnostic imaging examinations in England. Figures from the Department of Health show that the numbers of ultrasound scans (including obstetric and non-obstetric) more than doubled from 1996 to 2010.1 In fact, there were more ultrasound examinations performed than computed tomography (CT), magnetic resonance imaging (MRI), fluoroscopy and radio-isotope examinations combined.
Historically the use of ultrasound was confined to the radiology department, but advances in ultrasound technology made this modality more accessible to other healthcare professionals, resulting in a widening of its application across all fields of medicine: for example, obstetrics and gynaecology, general medicine, urology, orthopaedics, vascular studies, anaesthesia, paediatrics, etc.2–5 Sonography is not currently recognised in the UK as a profession in its own right and there is potential for this imaging modality to be misused. The use of ultrasound in diagnosis is highly operator dependent, and the greatest danger to a patient is the risk of diagnostic misinterpretation by an inadequately trained healthcare professional or the failure of the trained professional to maintain competencies. Not all sonographers are currently required/able to register with a regulatory body such as the Health Professions Council (HPC). However, the majority of practising sonographers are registered under their primary profession, for example radiographer, midwife, nurse or clinical scientist. The postgraduate training of these non-medical healthcare professionals in the UK has long since been standardised, with courses ratified by CASE (the Consortium for the Accreditation of Sonographic Education). In 2005 the Royal College of Radiologists (RCR) also published ‘Ultrasound training recommendations for medical and surgical specialties’ which set out the minimum standards to be achieved by non-radiological medical staff undertaking ultrasound scans as part of their working practice.6
Equipment chronology
1790 Spallanzani found that bats manoeuvre using hearing rather than sight.
1801 Young’s work on light shows that waves can be combined to become stronger or cancel each other out.
1826 Colladon determines the speed of sound through water.
1880 Pierre Curie discovers the piezoelectric effect in crystalline materials.
1917 Langevin invents the hydrophone. The device was able to send and receive low-frequency sound waves through water, and was used to detect submarines in World War I.
1936 Siemens launch the Sonostat, a therapeutic ultrasound machine that used the heating effects on tissue.7
Early 1940s Growth of use of A-mode ultrasound materials testing.
1942 Dussik publishes his work on transmission ultrasound of the brain; the first medical ultrasound publication?
Late 1940s Ludwig studies the difference in sound waves as they travel through various tissues in animals, later applying these findings to human subjects.
1949 Wild assesses the thickness of bowel tissue and pioneers early developments in ultrasound.8
1951 Donald produces static, black and white B-mode scanning.
1954 Edler and Hertz publish their work on measuring cardiac movement.9
1958 Donald’s equipment now able to demonstrate pathology in live volunteers. Publishes ‘Investigation of abdominal masses by pulsed ultrasound’.10
1962 First contact B-mode scanner developed, commercially launched in 1963.
1965 Advances in materials technology enable improvements in equipment and the development of real-time images.
1972 First linear array scanners available.
1973 Grey-scale B-mode available; developing computer technologies make ultrasound faster, with improving images.
1980s Fast real-time scanners become widespread, enabling wider range of hospital-based clinical applications.
1984 First 3D fetal ultrasound.
1985 Real-time colour flow Doppler.11
1990s Digital processing enables high-resolution imaging using broadband transducers. Image quality and improvements in accuracy further increase the role of ultrasound, particularly in breast imaging and cancer detection.
2000s 3D and 4D fetal and cardiac imaging becomes widespread; equipment featuring advanced system performance now widespread.
Physical principles
Ultrasound wave interactions
• Absorption. As the wave passes through the patient some of its energy is lost in the tissues through which it passes. The rate of absorption is dependent upon the tissue type and the frequency of the wave. The tissues are vibrated by the wave, producting heat. It is this heat that is used to advantage in therapeutic applications of ultrasound. In diagnostic applications the induced rise in temperature is a potential hazard.
• Reflection. The wave is reflected at tissue interfaces; some of these reflected echoes will return to the transducer, where they will induce an electric signal, forming the basis for the ultrasound image. The degree of reflection is dependent on the acoustic impedance mismatch at the tissue interface.
• Acoustic impedance mismatch. The acoustic impedance (Z) is a measure of how the wave traverses a particular tissue:
where v is the speed of sound in the tissue and ρ is the tissue density.

• Refraction. The wave may undergo refraction (change of direction) at a tissue interface if the wave front is not perpendicular to the interface. This phenomenon can cause misregistration and measurement artefacts.
• Diffraction. As the wave travels further from the transducer it becomes divergent, spreading the wave energy over a greater area and hence reducing its effective intensity.
Image formation
There are basic controls which can be used to optimise the ultrasound image. These include:
• Transducer frequency selection. A variety of transducers are available; on modern equipment they may be multifrequency. The higher the frequency of a transducer, the better the resolution, but at the expense of the depth of penetration.

• Time gain compensation. Amplifies signals that take longer to return (from deep interfaces) than those (from shallower interfaces) that return faster; this helps to counter the other effects of attenuation, which reduce the signal more from a more distant reflection.
• Overall gain. Amplifies all returning signals to provide a signal sufficient for display.
• Focus. Can be used to provide improved resolution of particular structures
• Dynamic range. Can be used to improve image contrast by manipulation of the displayed grey scale
Equipment and technology
There are a wide variety of ultrasound machines available commercially, from small handheld pieces to laptop-sized portable machines and on to the larger, static departmental machines (Fig. 38.2). It is essential that the correct machine is available to practitioners based on the case mix to be scanned, the throughput of patients expected and the location. It would be inappropriate to expect a sonographer to use a handheld device to scan a list of 20 patients on a machine not much bigger than a mobile phone, but these are ideal for use in ‘point of care’ situations where a large static machine would be impractical. Whenever machines are to be purchased, it is essential that sonographers are consulted to ensure that the most suitable equipment is acquired.
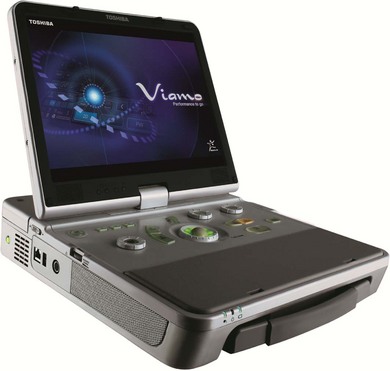
Figure 38.2 Image of portable ultrasound machine.
Reproduced with permission from Toshiba Medical Systems Ltd.
Room requirements and ancillary equipment
Where obstetric studies are undertaken a slave monitor is necessary to enable the parents to see the baby on screen without the sonographer having to scan in awkward positions, which has been shown to contribute to work-related upper limb disorder (WRULD) in the sonographer population.12
Imaging methods
Several different imaging methods are used in ultrasound imaging.
Doppler mode
• Continuous wave (CW) Doppler. Electronically, the more basic CW Doppler involves using a transducer with two crystals in a simutanoeus transmission and reception of ultrasound waves; the difference between the transmitted and received frequencies is measured. This dual function can present a disadvantage as there is no information regarding the depth of the vessel being examined or the velocity of the blood flow, and may cause some difficulties in the interpretation of the results, particularly if the ultrasound beam encounters more than one blood vessel along its path. Typical examples of CW Doppler applications are fetal heart monitoring and echocardiography.
• Pulsed wave (PW) Doppler. PW is used to measure the velocity and direction of flow. PW uses a transducer that detects the shift in frequency between transmission and reception that results from the moving targets. The degree of frequency shift depends on the velocity and direction of flow relative to the transducer, the transmitted frequency, and the speed of sound of the tissue investigated. The main advantage of a PW transducer is that the operator can select a specific vessel, usually from a grey-scale image of a colour Doppler, to be investigated, called the sample volume, thus returning echoes from vessels outside this chosen sample volume are eliminated.
• Colour Doppler imaging. Generally used when additional information is required, such as a pattern of flow within a conventional B-mode image, e.g. perfusion of a specific organ, neovascularity, the direction of blood flow, or to highlight regions of interest such as jets and stenoses. A colour box (sample volume) is placed over the region of interest, the resultant flow is colour coded and is calculated by positive Doppler shift, shown as red for flow towards the transducer, and negative Doppler shift shown as blue for flow away from the transducer. There may also be shades of orange and yellow, either where there is turbulent flow or the Doppler settings on the ultrasound equipment are not set correctly. The transducer alternates between B-mode and colour Doppler imaging, updating each image. This is also known as duplex imaging
• Power Doppler. Power Doppler maps the magnitude of the Doppler signal rather than the Doppler shift. Duplex imaging is used to superimpose a colour box onto a B-mode image, similar to colour Doppler. The resultant colour image is in shades of yellow, orange and red, depending on the strength of the Doppler signal (Fig. 38.3). This imaging mode is sensitive and therefore useful in detecting slow flow and flow through smaller vessels; however, unlike colour Doppler imaging there is no information on the direction of blood flow, and because of its sensitivity it is prone to motion artefacts.
Harmonic mode
In this mode a broad bandwidth transducer (i.e. a transducer capable of receiving a relatively wide range of frequencies) is used. A wave is transmitted at an initial, fundamental frequency, and interactions within the deeper tissues cause frequency shifts to harmonic frequencies. Harmonic frequencies are a multiple of the fundamental frequency. In harmonic imaging the information from the fundamental frequency is filtered out, therefore only the higher harmonic frequencies are used for image formation. Harmonic imaging offers several advantages over conventional imaging, including improved contrast resolution, reduced noise and clutter, improved lateral resolution, reduced slice thickness, reduced artefacts and improved signal-to-noise ratio (Fig. 38.4A,B). In general, harmonic imaging is useful when examining deeper structures and obese patients.13,14
Compound imaging
Real-time spatial and frequency compound imaging uses electronic beam steering to interrogate a structure from different viewing angles and at different frequencies. Several overlapping scans of the structure under investigation are obtained and averaged to form a multiangle compound image that is constantly updated in real time. Compound imaging improves image quality by reducing acoustic artefacts such as ‘speckle’, caused by coherent wave interference, and ‘clutter’, which can result from side lobes and reverberations. Applications include imaging of the breast, peripheral blood vessels and musculoskeletal injuries.14,15
Extended field of view (EFOV)
The advantage of EFOV is that larger organs or pathology can be seen in one single panoramic image. A real-time transducer is slowly swept along the area of interest, and successive images are interpreted and processed relative to the probe movement. It is most useful for superficial organs such as thyroid or breast, and for musculoskeletal imaging, where the entire length of a muscle and surrounding organs can be visualised (Fig. 38.5). Unfortunately, EFOV imaging is limited when there are other movements present apart from the transducer, e.g. fetal movements.15
Very high-frequency imaging and intracavity transducers
With the development of very high-frequency imaging the current imaging frequency range of between 1 and 15 MHz is increased by miniature transducers that will offer very high-resolution imaging at frequencies ranging from 20 to 100 MHz. These transducers can be placed within cavities to give high-resolution images of structures close to the cavity walls; examples of applications include transurethral, transoesophageal and intravascular scanning.14,15
Sonoelastography
Sonoelastography is an ultrasound technique for imaging the relative elastic properties of soft tissue and, in particular, for differentiating between benign and malignant tumours. Low-frequency (100–500 Hz) low-amplitude shear waves (a transverse wave that occurs when tissue is subjected to a change in shape without a change in volume) are transmitted into tissue and the resultant vibration is detected using colour Doppler. When a discrete hard mass, such as a tumour, is present in a region of soft tissue, a decrease in the vibration amplitude will occur at its location.14,15
3D and 4D ultrasound imaging
3D ultrasound uses a dataset that contains a large number of B-mode 2D planes. Once the volume data is obtained it is possible to optimise the ultrasound image of the area of interest by rotating, reconstructing and rendering, allowing viewing in different planes and angles without further exposure of the patient to ultrasound, thereby reducing scanning times. Unwanted information can be ‘sliced’ out and the stored data can then be recalled and manipulated after the ultrasound examination.16
4D ultrasound is also known as ‘real-time 3D ultrasound’. The basic concept is that the ultrasound equipment can acquire and display the 3D datasets with their multiplanar reformations and renderings in real time. However, 3D or 4D can only build on the 2D B-mode images, therefore the limitations and artefacts that affect B-mode imaging, such as presence of gas and overlying structures, will also affect the quality of the 3D and 4D imaging. The main advantage of 3D/4D is that this technique gives better visualisation of spatial relationships by multiplanar imaging, which is useful for therapeutic and follow-up examinations, and rendering abilities to convey information in a different manner (Fig. 38.6). Quantitative volume estimations can also be calculated more accurately than with conventional 2D scans. Despite these advantages, 3D and 4D ultrasound imaging is still considered a complementary tool rather than a replacement for 2D B-mode imaging.
Contrast-enhanced ultrasound (CEUS)
Ultrasound contrast agents are not only effective in ultrasonic imaging but are also important tools for the delivery of drug or gene therapy.17,18 Furthermore, when the use of CEUS is combined with Doppler and harmonic imaging, sensitivity is greatly increased. The application of CEUS is continuously evolving; however, it should be noted that the insonation of gas-filled microbubbles has the potential to cause a number of biological effects, for example the induction of a physiological response to cardiac exposures (premature ventricular contractions), and damage at a microvascular level (microvascular rupture and subcutaneous haemorrhage).19,20 The effect of insonation depends on the mechanical index (see section below on safety), the contrast agent used and the ultrasound imaging method. Although there is no proven evidence of harm resulting from clinical use of these agents, caution is recommended when contrast-enhanced imaging is undertaken.
Safety
It is important to highlight that although there is currently no absolute evidence that ultrasound imaging is harmful in humans, research has been carried out in laboratories and animal studies to investigate the effect of using high-intensity ultrasound.21 These studies have found that two main changes occur in body tissues:
• Thermal effect. There can be a localised rise in tissue temperature owing to the ultrasound energy being absorbed and converted into heat. This effect is displayed on the ultrasound monitor as a thermal index (TI). For example a TI of 1 indicates a temperature rise of 1°C. This is particularly important in obstetric scanning during development of the embryo and fetus. Three forms of the TI may be displayed:



• Cavitation effect. This can occur in the presence of very high ultrasound pressures, causing oscillation of microbubbles which can result in biological damage to tissue cells. The likelihood of cavitation occurring is related to the peak pressure and is referred to as the mechanical index (MI)
For all ultrasound imaging techniques, prudent use is advised. Comprehensive guidelines on the safe use of ultrasound have been developed by the Safety Group of the British Medical Ultrasound Society;22 these provide detailed advice on safe working levels for TI and MI. Adherence to these published guidelines and keeping exposure time ‘as low as reasonably achievable’ to produce an adequate image for interpretation and diagnosis will ensure that sonographers practise ultrasound imaging safely.
Health and safety of sonographers
The popularity of ultrasound and the reported increased prevalence of obese patients have affected the ultrasound workforce. The average time for sonographers to practise before experiencing work-related pain is 5 years.5 Inadequate equipment, environment and workload planning all have a considerable impact on the potential occupational hazard to the sonographer, especially in WRULD and musculoskeletal injuries to the associated muscles, tendons and ligaments caused by continuous movements of a repetitive, forceful or awkward nature. In addition, regular use of visual display units, such as the ultrasound monitor and reporting workstations, increases the potential for vision fatigue.
Guidelines have been developed by professional bodies5,23,24 after consultation with manufacturers, employers and sonographers to ensure appropriate working conditions and practices.
Clinical applications
Upper abdomen
Common clinical indications include:
The minimum images to be recorded should include:
• Left lobe of liver: sagittal section (SS) and transverse section (TS)
• Pancreas (ideally demonstrating head, body and tail): TS
• Right lobe of liver: SS/TS. The size of the liver is based on a measurement from the right hemi-diaphragm to inferior border in the midclavicular line
• Right lobe of liver: through the porta hepatis
• Common bile duct: measurement at widest point
Common pathology
The liver parenchyma is normally homogeneous and of a similar reflectivity to the renal cortex. Focal lesions can be readily identified, measured, and their blood flow assessed using Doppler. Diffuse pathology such as cirrhosis and fat infiltration, and focal lesions such as simple cysts, haemangiomas and metastases, can usually be confirmed or excluded (Fig. 38.7A,B).
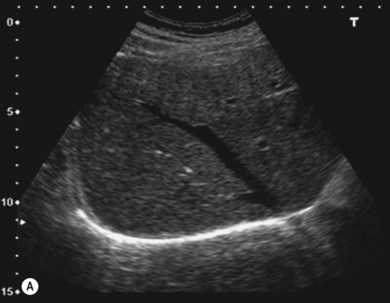
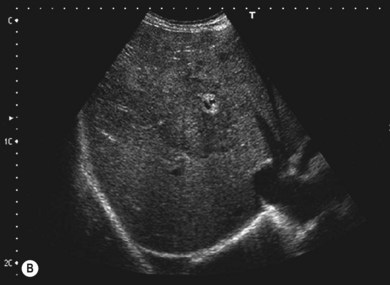
Figure 38.7 (A) Normal liver showing right hepatic vein; (B) liver with tumour demonstrated.
Reproduced with permission from Toshiba Medical Systems Ltd.