Abstract
Objective
Ultrasound in combination with microbubbles can enhance accumulation and improve the distribution of various therapeutic agents in tumor tissue, leading to improved efficacy. Understanding the impact of treatment on the tumor microenvironment, concurrently with how microenvironment attributes affect treatment outcome, will be important for selecting appropriate patient cohorts in future clinical trials. The main aim of this work was to investigate the influence of ultrasound and microbubbles on the functional vasculature of cancer tissue.
Methods
Four different tumor models in mice (bone, pancreatic, breast and colon cancer) were characterized with respect to vascular parameters using contrast-enhanced ultrasound imaging. The effect of treatment with microbubbles and ultrasound was then investigated using immunohistochemistry and confocal microscopy, quantifying the total amount of vasculature and fraction of functional vessels. Two different microbubbles were used, the clinical contrast agent SonoVue and the large bubbles generated by Acoustic Cluster Therapy (ACT), tailored for therapeutic purposes.
Results
The colon cancer model displayed slower flow but a higher vascular volume than the other models. The pancreatic model showed the fastest flow but also the lowest vascular volume. Ultrasound and SonoVue transiently reduced the amount of functional vasculature in breast and colon tumors immediately after treatment. No reduction was observed for ACT, likely due to shorter ultrasound pulses and lower pressures applied.
Conclusion
Variation between tumor models due to tissue characteristics emphasizes the importance of evaluating treatment suitability in the specific tissue of interest, as altered perfusion could have a large impact on drug delivery and therapeutic outcome.
Introduction
Microbubbles in combination with ultrasound have shown promise for enhancing local delivery of chemotherapeutic drugs, nanomedicines, checkpoint inhibitors, genes and other therapeutic agents to tumor tissue in pre-clinical [ ] and clinical studies [ , ]. The microbubbles are administered intravenously, and can be either loaded or co-administered with the therapeutic agent. Both clinically approved microbubbles, commonly used for diagnostics, and microbubbles tailored for therapeutic applications [ , ] have been used. During sonication, the microbubbles create biomechanical effects such as pushing and pulling on the vessel wall, shear stresses through microstreaming, shock waves and microjets, which can increase the permeability of vessel wall, extracellular matrix and cell membranes [ ]. Biomechanical effects on the tissue will depend on ultrasound parameters such as frequency, pressure amplitude, pulse length and treatment duration, as well as microbubble size, gas and shell properties. To understand how microbubbles and ultrasound parameters can be tuned for optimal efficacy, it is crucial to understand how the treatment influences tumor vasculature and perfusion. To assess which patients and which tumors could benefit from such treatment, we also need to characterize how vascularization impacts the outcome of ultrasound-mediated drug delivery. Tumor vasculature is important for the distribution of both therapeutic agents and microbubbles, in addition to nutrients and oxygen in the tissue. Tumor vasculature is known to be dilated and highly heterogeneous, with a tortuous and disorganized structure, as well as collapsed vessels and slow or even alternating blood flow [ ].
The impact of ultrasound and microbubbles on tumor vasculature has been described previously. Reduced perfusion after treatment was observed in various pre-clinical tumor models, including melanoma [ ], prostate cancer [ ], colon cancer [ ], breast cancer [ ], liver cancer [ ] and glioma [ ]. However, increased vascularization or perfusion has also been reported in colon cancer [ ], pancreatic cancer [ , ], breast cancer [ ] and prostate cancer [ ]. The effect of ultrasound and microbubbles on tumor vasculature appears to depend on the type of tissue, the type of microbubble and the ultrasound parameters used. The aim of the present work was to study whether ultrasound and microbubbles would affect perfusion differently in tumors with different vascular density and distribution of the vasculature. Thus, the vascular parameters of four tumor models in mice were characterized using contrast-enhanced ultrasound (CEUS) imaging. The models were murine breast cancer (4T1), colon cancer (CT26), pancreatic cancer (KPC) and human osteosarcoma (OHS), all grown subcutaneously in the hind leg of mice. We also hypothesized that microbubbles designed for ultrasound imaging, with a typical diameter of 2–3 µm and a circulation time of a few minutes [ ], would affect perfusion differently compared with large microbubbles with a diameter of 10–40 µm and circulation time of 5–10 min [ , ] designed for ultrasound-mediated drug delivery. Therefore, the tumors were treated with either the commercial SonoVue or Acoustic Cluster Therapy (ACT) using ultrasound parameters previously shown to enhance the uptake and therapeutic efficacy of free and encapsulated chemotherapies [ , , , , , ]. ACT is based on intravenous injection of microclusters consisting of negatively charged microbubbles and positively charged oil microdroplets. Upon ultrasound exposure the oscillating microbubbles transfer energy to the microdroplets, leading to instant vaporization of the microdroplets forming large bubbles that transiently lodge in a fraction of the microvasculature (activation step) [ , , ]. A second insonation at lower frequency induces volume oscillations of the ACT bubble (enhancement step), which induce biomechanical effects on the vessel wall, increasing local vascular permeability [ , , ]. All four tumor models were treated with ultrasound and SonoVue, whereas KPC and CT26 tumors were treated with ACT, as these two tumor models have different extents of vascularization. KPC tumors are generally poorly vascularized and vessels mainly occur in the tumor periphery [ ], whereas CT26 tumors are vascularized throughout the whole tumor [ ]. After treatment with SonoVue or ACT, fluorescently labeled lectin was injected intravenously to stain functional blood vessels. The tumors were then excised and sectioned, and immunohistochemistry was applied to stain all vasculature in the tissue. All blood vessels and functional vessels were imaged by confocal microscopy to characterize the fraction of functional vasculature in treated and untreated tumors.
Materials and methods
Cell culture
The OHS cell line (Radium Hospital, Oslo, Norway) [ ] was cultured in Roswell Park Memorial Institute (RPMI) 1640 medium (R0883, Sigma-Aldrich, MO, USA). Murine colorectal CT26 (CRL-2638, American Type Culture Collection (ATCC), VA, USA) and breast 4T1 (CRL-2539, ATCC) cancer cells were cultured in RPMI 1640 medium (30-2001, ATCC). KPC cells were isolated from pancreatic ductal adenocarcinoma tumors in transgenic KPC mice (Department of Radiation Oncology, Massachusetts General Hospital, MA, USA) and cultured in Dulbecco’s Modified Eagle Medium (Gibco 11960-044, Thermo Fisher Scientific, MA, USA). All cell culture media were supplemented with 10% fetal bovine serum (F7524, Sigma-Aldrich) and 1% penicillin-streptomycin (P0781, Sigma-Aldrich), while the media for OHS and KPC also contained 0.5% l -glutamine (G7513, Sigma-Aldrich). The cells were maintained in exponential phase at 37°C with 5% CO 2 . Before implantation, they were detached by trypsination, counted and resuspended to the desired concentration in the respective cell culture media. The cells were stored on ice until implantation in mice.
Animal and tumor implantation
All experimental procedures were approved by the Norwegian Food Safety Authorities and conducted according to recommendations of the Federation of European Laboratory Animal Associations. Mice were purchased at 8 wk of age (Janvier, Le Genest-Saint-Isle, France). They were housed in groups of five in individually ventilated cages under specific pathogen-free conditions. The cages were enriched with bedding, housing, nesting material and gnaw sticks, and were kept in a controlled environment (22–23°C, 50%–65% humidity, 65 air changes/h) at a 12-h day/night cycle. Animals had free access to food and sterile water. During all experiments, the animals were anesthetized by inhalation of 2%–3% isoflurane (Baxter, IL, USA) in 0.4 L/min O 2 and 0.6 L/min N 2 O during cell implantation, 1 L/min O 2 during ultrasound imaging and 1 L/min medical air during ultrasound treatment. Body temperature was maintained by heating lamps or heating pads, and eye gel (Viscotears, Alcon, Geneva, Switzerland) was used to keep the eyes moist. Subcutaneous tumors were grown from all four cancer cell lines by injection of 50 µL cell suspension per animal. Three million OHS cells were implanted in the hind leg of BALB/c nude mice (n = 19). For CT26 and 4T1, 100 000 and 10 000 cells, respectively, were injected subcutaneously into the hind leg of BALB/c mice (n = 26 and 35, respectively). A total of 200 000 KPC cells were injected subcutaneously into the hind leg of a B6/albino mice (n = 35). The number of animals was based on previous experience with the same tumor models and their tumor take, and included five to seven mice per group. The tumors were measured using calipers, and experiments were performed when the tumors were 6–9 mm in the longest direction, around 2–3 wk after implantation. A tail vein catheter (24 GA BD Neoflon, Becton Dickinson, NJ, USA) was placed for intravenous injection of microbubbles and fur was shaved on the tumor leg to obtain good contact with the ultrasound through gel or water.
Tumor stiffness measured using indentation
Young’s modulus of KPC and CT26 tumors were measured using microindentation. Tumors were placed into petri dishes with phosphate-buffered saline (PBS; Sigma-Aldrich) to prevent dehydration. Large tumors were cut in two, whereas small tumors were kept intact. A stepper motor (TRB25CC, Newport, CA, USA) was combined with a custom-made 1 mm spherical indenter, i.e. , radius 0.5 mm, and a scale (YAC01ED, Sartorius, Göttingen, Germany). Data were acquired and stored using a custom-made LabView (National Instruments, TX, USA) script. Indentation was performed at 2 μm/s for approximately 200 μm at five different positions on the tumor. The force indentation curves were fitted to the Hertz model, as previously described [ ], to extract Young’s modulus.
CEUS imaging and analysis
MicroMarker (FUJIFILM VisualSonics, Toronto, Canada) were reconstituted according to protocol from the supplier, by adding 0.7 mL saline to the vial, followed by gentle agitation for 1 min. The animal was then positioned on the stage for imaging by Vevo 3100 (FUJIFILM VisualSonics) using an MX250 probe at 18 MHz and a frame rate of 1 fps. Heated ultrasound gel (ECO Supergel, Ceracarta, Forli, Italy) was added to the leg of the animal before the probe was placed to image the cross-section of the largest diameter of the tumor. Before injection, a 3D scan of the tumor was recorded with a step size of 152 µm. A 2D recording at the largest diameter of the tumor was started and 50 µL MicroMarker (1 × 10 8 microbubbles) was injected intravenously followed by imaging for approximately 6 min. A new 3D scan was then recorded before the catheter was removed and the animals were woken up and placed back in the cage until the next day.
Analysis of the CEUS 2D bolus videos was performed using the module VevoCQ in VevoLab (FUJIFILM VisualSonics). For each tumor, regions of interest (ROIs) were drawn manually to delineate the tumor tissue in B-mode and non-linear contrast mode ( Supplementary Figure S1 ). For each ROI in non-linear contrast mode the “quantify” tool was used to extract peak enhancement, rise time, wash-in area under the curve and wash-in rate, which are indicators of blood volume and flow of the microbubble bolus injection. Representative examples of 2-D time–intensity curves are shown in Supplementary Figure S2 . Subsequently, the perfused area was quantified using ImageJ (version 1.51c, National Institutes of Health, MA, USA). Two images were extracted and converted to 8-bit grayscale images, one pre-injection and the other at the time of peak enhancement. The pre-injection image was subtracted from the peak enhancement image before an ROI was drawn around the tumor. The signal inside the tumor was thresholded using the algorithm called “default,” which best represented the observed microbubble signal. The area of the ROI and the percentage of the area covered with microbubbles were measured. The 3-D scans were analyzed using VevoLab to extract tumor volume and perfused volume of the tumors. Multiple ROIs were drawn through the stack using the parallel volume measurement. To estimate the perfused volume, pre-injection perfused agent (PA) values were subtracted from the post-injection PA values. Maximum intensity projection images were also created using VevoLab to distinguish between highly and poorly perfused areas.
Microbubbles and ultrasound treatment
For ultrasound treatment the mouse was positioned on top of a water tank with the tumor-bearing leg lowered into the water through a 10 mm opening in the lid, as described previously [ , ]. The surface of the lid was covered with an absorbing material to avoid standing waves (polyester wadding of 7 mm thickness). A waveform generator (33500 B, Keysight Technologies, CA, USA) and a 50 dB power amplifier (2100 L, Electronics and Innovations Ltd., NY, USA) were used to generate the ultrasound waves. The water in the tank was heated to 34°C and a heating lamp was used from above to avoid hypothermia in the animals.
SonoVue (Bracco, Milan, Italy) was reconstituted according to protocols from the supplier by injecting 5 mL sodium chloride (0.9%) to the vial, followed by shaking for 20 s. A custom-made single-element focused ultrasound transducer with a center frequency of 1 MHz was used (Imasonic, Voray sur l’Ognon, France). The geometric focus of the transducer was at 12 cm, but the tumor was positioned in the far field at 19 cm to ensure sonication of the entire tumor volume. The transducer was calibrated in an Onda AIMS III water tank with an HGL-0200 hydrophone (Onda Corp., CA, USA), and the beam widths at 19 cm were found to be 6 and 10 mm at 3 and 6 dB, respectively. The input voltage to the transducer was monitored using an oscilloscope (WaveSurfer 44Xs, LeCroy, NY, USA). The tumors were sonicated using a pulse length of 10 ms (10 000 cycles) and a peak negative pressure of 0.5 MPa (mechanical index (MI) 0.5). A pulse repetition frequency of 0.25 Hz was applied (duty cycle 0.25%) and the total treatment time was 9 min, during which 50 µL SonoVue was administered at 0, 3 and 6 min. These parameters were previously shown to induce both stable cavitation and bubble destruction, and increased delivery of both free and nanoparticle-encapsulated drugs to tumors [ , ]. Animals in the control groups received injections of saline or microbubbles but no ultrasound.
ACT microclusters were prepared by reconstituting a freeze-dried suspension of commercially available negatively charged microbubbles of perfluorobutane (Sonazoid powder for injection, 16 µL/vial) with 2 mL of a positively charged microdroplet emulsion of perfluoromethylcyclopentane (6.8 mg/mL) stabilized by a distearoylphosphatidylcholine phospholipid membrane containing 3% (mol/mol) stearyl amine, dispersed in a 5 mM TRIS buffer. Sonazoid powder for injection and perfluoromethylcyclopentane emulsion were supplied by GE Healthcare AS and EXACT Therapeutics (Oslo, Norway), respectively. A custom-made dual-frequency ultrasound transducer [ ] was used for ACT, operating at the fundamental 0.5 MHz and fifth harmonic 2.7 MHz. The tumor was positioned at 20 cm, just beyond the pressure maximum, where the beam widths were 16 and 6 mm, respectively [ ]. First, the tumors were sonicated using an activation sequence at 2.7 MHz, with a pulse length of eight cycles, a pulse repetition frequency of 1 kHz, and an MI of 0.24 for a total of 45 s. Subsequently, the enhancement step at 0.5 MHz was applied, with a pulse length of two cycles, pulse repetition frequency of 1 kHz, an MI of 0.2 and a total treatment time of 5 min. ACT was administered three times, with 50 µL of microclusters injected in each cycle before consecutive sonications. These settings were described previously to enhance uptake and therapeutic efficacy in tumors [ , , , ]. Animals in the control groups received injections of saline or microbubbles but no ultrasound.
Tumor sectioning and immunohistochemistry
To stain functional vessels, 50 µL fluorescein (FITC)-labeled Lycopersicon esculentum tomato lectin (Vector Laboratories, CA, USA, 2 mg/mL) was injected intravenously, either immediately or 1 h after treatment. After 5 min of circulation, the anesthetized mice were euthanized by cervical dislocation. The tumors were removed, placed on cork with Tissue-Tek O.C.T. Compound (Sakura, Alphen aan den Rijn, Netherlands) and frozen in liquid nitrogen. They were stored at -80°C until sectioning. The tumors were sectioned into 25, 8 and 5 µm sections, of which selected 5 and 8 µm were stained with hematoxylin, erythrosine and saffron (HES).
Immunohistochemistry was performed to stain all vessels, both functional and non-functional vasculature, in frozen tumor sections from the different tumor models. Briefly, the 8 µm sections were thawed and washed in PBS for 5 min, fixed for 5 min with acetone (Sigma-Aldrich), and washed again with PBS for 5 min. The tumor section was then delineated using a PAP Pen (Sigma-Aldrich). Blocking of unspecific binding of antibodies was done by incubating the section in 12% bovine serum albumin (BSA; Sigma-Aldrich) in PBS for 50 min, before washing again with PBS. Anti-CD31, which stains endothelial cells (purified rat anti-mouse CD31, BD Pharmingen, NJ, USA), was diluted with BSA-PBS at a ratio of 1:50 (0.01 mg/mL) and incubated on the section for 1 h at room temperature, before washing with PBS three times for 5 min. The secondary antibody used was either Alexa Fluor 647 AffiniPure donkey anti-rat IgG (Dianova, Hamburg, Germany) or Cy3 AffiniPure Donkey Anti-Rat IgG (Jackson ImmunoResearch Europe Ltd, Ely, UK). The secondary antibody was diluted 1:50 (AF647, 0.03 mg/mL) or 1:500 (Cy3, 0.0014 mg/mL) with BSA-PBS and incubated on the section for 1 h in the dark at room temperature. Washing with PBS was repeated before the sections were mounted using VectaShield Vibrance (Vector Laboratories) and a cover slip, and stored in the dark at 4°C until imaging. A similar protocol was applied for 25 µm sections, except fixation was performed using methanol for 5 min and acetone for 2 min, and a permeabilization step was included using Triton X-100 (0.1%, Sigma-Aldrich) for 10 min before subsequent staining with antibodies.
Confocal imaging and image analysis
Imaging the frozen tumor sections was performed using a confocal laser scanning microscope (LSM 800 Airyscan, Carl Zeiss AG, Oberkochen, Germany) with a plan-apochromat 20×/0.8 objective. One section per tumor was imaged, except for OHS tumors where three sections were imaged per tumor and the average value per tumor was displayed. A 488 nm laser was used to excite FITC, which was detected at 488–560 nm. A 561 nm laser was used to excite Cy3, which was detected at 561–700 nm, and a 640 nm laser was used to excite AF647, which was detected at 656–700 nm. A dichroic beam splitter suppressed the lasers, allowing only emission light to enter the detectors. The laser intensity and detector gain were optimized to achieve the best signal with minimal background noise and saturation. Tile images of the entire tumor sections were captured, stitching adjacent frames of 512 × 512 pixels together. For each tumor model, the same settings were used to image treated and non-treated sections. Tile scans of the HES–stained sections were acquired using a Zeiss LSM 800 microscope in bright-field mode using a plan-apochromat 20 × 0.8 NA or 10 × 0.45 NA water immersion objective. These were used to delineate the tumor boundary ( Supplementary Figure S3 ).
To quantify the amount of functional and all blood vessels, confocal laser scanning microscopy (CLSM) tile scans were analyzed using Fiji (Fiji Is Just ImageJ, version 2.9.0/1.53t). The Cy3 or AF647 channel (red channel) and the FITC channel (green channel) were split. The red channel was processed first and used as a mask for the green channel. A median filter of two pixels was applied to remove background noise. Gray morphology with a closing diamond structure of radius three pixels was used to compensate for disrupted vessels. Thresholding of the red channel was then performed using Fiji’s “Li” algorithm to create binary images, with threshold values between 18 and 22, creating a mask. The mask was used to remove signal from the green channel that was outside the vasculature, such as background autofluorescence. The green channel image was then processed using the same method as for the red channel, but with threshold values between 8 and 14, making binary images. Examples of the thresholded red and green channel, in addition to their overlay, can be seen in Supplementary Figure S4 .
Next, the tumor was divided into three different ROIs—the entire tumor, the center and the periphery including only the outer 10% of the longest tumor diameter ( Supplementary Figure S3 ). The total amount of blood vessels was found by measuring the area fraction in the red channel, and the amount of functional blood vessels by measuring the area fraction in the green channel. The researcher who performed the analysis was blinded to which group the animals belonged.
Statistical analysis
Statistical analysis and plotting of the results were performed using Prism (Version 9.5.0 [525], GraphPad Software, LLC, MA, USA). To compare whether the control and treated animal groups were significantly different, unpaired t -tests were performed. A Shapiro-Wilk test was used to test for normality. To compare more than two groups, a one-way analysis of variance was used when groups displayed normal distribution. If the data did not pass the normality test, a Kruskal-Wallis test was performed. Any statistically significant differences are indicated in the plot (* for p values < 0.05, ** for p values < 0.01, *** for p values < 0.001 and **** for p values < 0.0001).
Results
CEUS characterization of tumor perfusion
The perfusion parameters obtained from CEUS imaging are shown in Figure 1 . Peak enhancement was similar between the four different tumor models. Rise time increased from KPC, to 4T1, to OHS and CT26, indicating that CT26 has the slowest blood flow of the four models. The same trend can be seen in the wash-in area under the curve. The wash-in rate showed the opposite trend, although not statistically significant, decreasing from KPC, to 4T1 and OHS, and to CT26. KPC, 4T1 and OHS displayed similar perfused area, and CT26 was significantly higher, indicating that CT26 has the highest amount of vasculature/blood volume. The CT26 tumors also had a higher perfused volume than the other models, quantified 6 min after the bolus injection. The volume of the tumors was in the range of 363–508 mm 3 (363 ± 63 mm 3 for KPC, 490 ± 86 mm 3 for 4T1, 508 ± 146 mm 3 for CT26 and 492 ± 193 mm 3 for OHS).
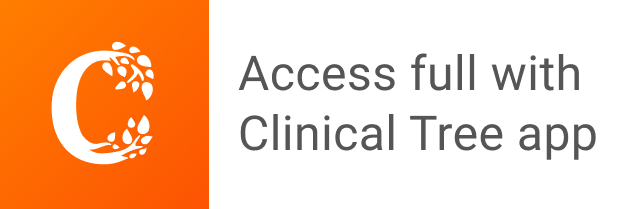