14
Vascular Brachytherapy
Chien Peter Chen, Ray Lin, and Prabhakar Tripuraneni
Vascular disease is the number one cause of mortality and morbidity in the United States. Cardiovascular disease accounts for approximately 1 million deaths annually. The most common pathology of vascular disease is an occlusive or stenotic process due to atherosclerotic plaque. Approximately 1 million coronary angioplasty procedures are performed annually in the United States. The magnitude of the impact to society due to peripheral vascular disease (PVD) is also significant compared with that of heart disease. Currently, more than 400,000 vascular interventions are performed in the United States annually for PVD (including atrioventricular [AV] graft for dialysis).
Over the past few decades, major improvement in the management of vascular disease has been made, with one of the most significant contributions being the development of percutaneous vascular recanalization and balloon angioplasty. The main concept behind the percutaneous transluminal angioplasty (PTA) procedure is that the atherosclerotic plaque can be physically removed by a specially designed catheter. Within a few years of its introduction, the minimally invasive PTA became the well-established standard for many vascular disorders previously treated with invasive surgery. Unfortunately, long-term efficacy of PTA is limited by the high rate of excessive wound healing causing restenosis. Restenosis is common, morbid, and expensive. It also limits the number of vascular interventions to be performed. Initial therapeutic approaches focused on pharmaceutical agents, mechanical devices (atherectomy or tissue removing), and physical devices (stenting). The placement of coronary stents has significantly decreased the incidence of restenosis from approximately 40% to approximately 20% to 25%, but there remains a significant population of patients each year who present with in-stent restenosis of coronary arteries. These patients have proven to be a therapeutic challenge for the medical community.
Over the last 10 years, multiple prospective randomized trials have been performed to show that risk of restenosis is reduced by radiation therapy. The U.S. Food and Drug Administration (FDA) approved three delivery systems for endovascular brachytherapy for use in patients with in-stent restenosis in native coronary arteries in 2002. These trials led to FDA approval of the Checkmate device using iridium-192 (192Ir) by Cordis Corporation, the BetaCath device using strontium-90 (90Sr) by Novoste Corporation, and the Galileo device using phosphorus-32 (32P) by Guidant Corporation for use in patients with in-stent restenosis in native coronary arteries. The use of vascular brachytherapy (VBT) exploded onto the scene of interventional cardiology and was quickly incorporated into the mainstream of interventional cardiology clinical practice. VBT was performed in approximately 100,000 patients at 300 centers across the United States in 2002. However, in the last few years, VBT has been replaced by drug-eluting stents (DESs).
Although enthusiasm for VBT has decreased with the advent of DES therapy, the evolution of VBT into the practice of interventional cardiology carries significant historic value. This chapter describes the basic pathophysiology, major clinical trials, treatment planning approaches, and future perspectives for VBT.
NORMAL ARTERY ANATOMY
A typical artery consists of three concentric zones or layers, which are intima, media, and adventitia (Figure 14.1). The intima extends from the endothelial lining to the internal elastic lamina (IEL). The intima consists of the endothelium, subendothelial connective tissue, basal lamina, and IEL. The endothelial cells regulate several important processes such as coagulation, platelet aggregation, fibrinolysis, leukocyte adhesion, and cell migration. The endothelial cells provide a system of checks and balances that control the final thrombotic–fibrinolytic state. Subacute and late thrombosis after VBT may be a consequence of crush injury and radiation-induced injury to the endothelial cells secondary to the disruption of this delicate system, leading to abnormal thrombotic–fibrinolytic events.
The media layer extends from the IEL to the external elastic lamina (EEL). In some vessels, the EEL is not indistinct from the adventitia. The media consists mostly of smooth muscle cells (SMC). The main function of the SMC is to maintain vascular tone. When the vessel wall is injured by angioplasty, the macrophages and other cells can “turn on” the SMC from a quiescent state into synthetic, migratory, and proliferative states. This chain reaction can lead to neointimal hyperplasia. Because the macrophages are sensitive to radiation, they can be inactivated by low-dose radiation. This simplified explanation is the basis for the use of radiation in preventing restenosis.
Figure 14.1 Cross-section of the layers of the coronary artery.
PATHOPHYSIOLOGY
Postangioplasty restenosis is a hyperproliferative response to tissue injury. A cascade of events due to altered local hemodynamics and endothelial injury leads to this hyperproliferative, excessive, and uncontrolled growth.
The pathophysiology of restenosis after coronary artery intervention is a multifactorial process that consists of vascular injury secondary to intervention followed by the physiologic response to the injury. Injury occurs in the intimal and medial layers, but the response to this injury occurs in the medial and adventitial layers. The vascular restenosis process can be divided into early and late phases. The early phase of restenosis involves vessel recoil that can result in an acute decrease in lumen diameter. The cause is physical stretching and contraction of injured or torn segments in the treated vascular region. The late phase of restenosis involves late vessel remodeling, which results in contracture of the EEL. This leads to neointimal hyperplasia, the growth of myofibroblasts and extracellular matrix that narrows the vessel lumen (1).
Stenting during the angioplasty procedure has led to a significant decrease in restenosis rates following angioplasty. However, in-stent restenosis persists as a significant and difficult clinical problem. It is believed that stenting at the time of angioplasty prevents vascular recoil and contracture of the vessel lumen but does not prevent neointimal proliferation. VBT targets this aspect of the restenosis process. It is believed that the adventitial layer is the main source of cells that produce neointimal proliferation. Adventitial myofibroblasts are the main target of ionizing radiation (2).
Low-dose ionizing radiation has been utilized for decades to treat nononcologic hyperproliferative disorders that include arteriovascular malformation (AVM), heterotopic ossification (HO), gynecomastia, keloids, and pterygium (3). The molecular and cellular basis of the antiproliferative action of radiation therapy in these benign conditions has been extensively investigated both in animal studies and clinical trials. Similar to these studies, the cellular and molecular targets of ionizing radiation in reducing vascular restenosis have been extensively studied. Although the injury from angioplasty and stenting occurs in the intimal and medial layers, it is the proliferative response in the medial and adventitial layers of the vessel lumen that leads to restenosis. It is believed that this overly exuberant response to arterial wall injury after angioplasty results in approximately 40% of patients exhibiting excessive healing and restenosis after angioplasty. Prosthetic stent implantation has significantly reduced the incidence of restenosis (4). However, a restenosis rate of 20% to 25% persists (5). Even modest doses of ionizing radiation therapy reduce in-stent restenosis rates by targeting the reproductive ability of the myofibroblast precursor cell and disrupting a complex anti-inflammatory and wound-healing response.
CORONARY ARTERY ANATOMY AND INTERVENTIONAL CARDIOLOGY TERMINOLOGY
Coronary Anatomy
The left and right coronary arteries, which arise from the left and right aortic sinuses, provide the major blood supply to the heart. They course in an epicardial manner and then terminate in a capillary network, forming a ring-loop system in two orthogonal planes. The right coronary artery and the left circumflex (LCx) artery course along the AV groove and form a circle between the atria and ventricles, whereas the left anterior descending (LAD) and the posterior descending right coronary artery form a half-circle along the interventricular groove and encircle the left ventricular apex (Figure 14.2).
The left main coronary artery originates from the left sinus of Valsalva and quickly branches to give rise to the LAD and LCx arteries. The LAD wraps around the apex of the left ventricle in most patients and gives rise to septal, diagonal, and other branches that extend to the right ventricular wall. The LCx artery follows the same direction as the left main to enter the left AV grove, and then it moves away from the LAD. The LCx gives off the obtuse marginal branches proximally, then it wraps around the posterior of the heart and gives off the posterolateral (PL) branches. The right coronary artery arises from the right sinus of Valsalva and can be divided into the proximal, mid, and distal segments. It gives rise to the infundibular artery, which provides blood to the right ventricular outflow tract, the sinus node artery, and the AV nodal artery. The right coronary artery also gives rise to acute marginal branches, which supply the right ventricular wall, the posterior descending artery, and several PL branches.
Figure 14.2 Anatomic layout of coronary arteries.
Interventional Cardiology and Restenosis Terminology
The world of interventional cardiology is one of acronyms and this section is included to assist in understanding the literature (6).
Angioplasty: A nonsurgical treatment for obstructive coronary artery disease (CAD) using a miniaturized balloon catheter to dilate obstructive lesions in coronary vessels.
Coronary artery bypass grafting (CABG): A surgical technique to improve myocardial blood flow. Arterial or venous conduits are used to bypass obstructive coronary lesions and reroute blood flow to the left ventricular myocardium.
Diameter stenosis: This term refers to the percentage of obstruction of a coronary vessel as determined by quantitative coronary angiographic analysis. The percent diameter stenosis is calculated by measuring the vessel diameter at the level of highest degree of obstruction and comparing it to the normal reference vessel diameter (RVD). The following equation illustrates the determination of percent diameter stenosis:
where MLD = minimal lumen diameter.
Acute gain: A term used to characterize the acute results of percutaneous coronary revascularization (PCR) procedures. The initial or acute gain represents the change in luminal diameter at the location of the target lesion undergoing revascularization. The equation used to calculate initial or acute gain follows:
Initial or acute gain (mm) = MLDpostprocedure – MLDpreprocedure
Late loss: A term used to describe the change over time in the vessel lumen diameter of a lesion treated with percutaneous coronary interventional (PCI) procedures. This term characterizes the tendency toward a loss of some of the initial gain achieved during PCI procedures. The following equation illustrates the method used to calculate late loss:
Late loss (mm) = MLDpostprocedure – MLDfollow-up
Late loss index: A term that reflects the loss over time of the initial gain achieved with PCI procedures. The loss index is calculated as follows:
Edge effect (candy wrapper effect): Restenosis of a previously irradiated lumen at the edges of the target volume (TV). This effect is felt to be secondary to hyperplastic changes and negative remodeling at the edges of the irradiated segment.
Major adverse cardiac events (MACE): This term is used to describe a composite of various end points used in interventional cardiology trials to describe outcomes. MACE varies among different trials and common components of the end point include death, myocardial infarction, repeat revascularization, and repeat hospitalization.
Minimal lumen diameter (MLD): This is a quantitative coronary angiography (QCA) term used to describe the diameter in millimeters of a target vessel undergoing PCI. The MLD is the smallest measurement of lumen diameter at the site of intervention in the target vessel. The MLD before and after PCI procedures is used to assess the acute and long-term success rates of percutaneous revascularization procedures.
Percutaneous coronary interventional (PCI): This term is used to describe in detail all percutaneous revascularization procedures including angioplasty, atherectomy, and other techniques used to accomplish catheter-based revascularization therapy.
Percutaneous coronary revascularization (PCR): This term is essentially identical to PCI and reflects a composite term used to describe all catheter-based PCR techniques and procedures.
Percutaneous transluminal coronary angioplasty (PTCA): This term is synonymous with balloon angioplasty and is used interchangeably as an acronym.
Quantitative coronary angiography (QCA): This is a technique used to analyze the acute and long-term results of PCR procedures. Computer-based techniques using automated edge detection systems are used to measure coronary vessel diameters and calculate percentage obstruction of atherosclerotic lesions. QCA techniques are used in interventional cardiology trials to assist in determining the incidence of restenosis following PCI procedures.
Reference vessel diameter (RVD): This measurement assists in characterizing the severity of obstruction in a coronary vessel containing an atherosclerotic lesion. The RVD (mm) is the maximum dimension of the vessel in a normal-appearing segment adjacent to a significantly obstructive lesion. The RVD allows calculation of the percent diameter stenosis.
Table 14.1 Target volume definitions for VBT
GTV | Stenotic or restenotic lesion |
CTV | Intervened or injured (angioplasty, stent, stent deployment, atherectomy) length |
PTV | CTV + uncertainty for heart/catheter movement + uncertainty in target localization |
TV | PTV + penumbra effect |
CTV, clinical target volume; GTV, gross target volume; PTV, planning target volume; TV, target volume; VBT, vascular brachytherapy.
Target lesion revascularization (TLR): A term used in the clinical definition of coronary restenosis. TLR is repeat revascularization of a lesion due to recurrent obstruction at the site of prior revascularization.
Target vessel revascularization (TVR): This refers to revascularization of a vessel that previously underwent PCI. This term is inclusive of TLR and occurrence of obstructive lesions requiring revascularization at other sites within the target vessel.
VBT TERMINOLOGY
On the basis of International Commission of Radiation Units and Measurements (ICRU) 62, terminology for VBT volumes was proposed taking into consideration both radial and longitudinal dimensions (7). This terminology will help define the TV with the attention to appropriate margins to decrease edge failures. The gross target volume (GTV) is the length of stenotic segment with appropriate radius, which may vary during the length. The clinical target volume (CTV) is the interventional length, which is delineated by the most proximal and most distal extents of injury and is always larger than GTV. CTV is often asymmetric from GTV or may be more on one end than the other. The planning target volume (PTV) is the CTV plus a margin to account for both the heart and catheter movements and inaccuracies in the visual delineation of the ends of CTV. The uncertainty or magnitude of the margin depends on the location of the target within the vessel, the delivery system (centered or noncentered), and the cardiac cycle. The TV is the volume irradiated on the basis of the PTV and the penumbra of effect of the isotope, which depends on the isotope, source design, and the prescription distance (Table 14.1).
HISTORICAL PERSPECTIVE
Coronary VBT
γ-Emitting Sources
VBT was tried in Germany by Liemann et al on restenosed femoral popliteal arteries in 1990 with no long-term adverse events noted at 10 years (8). The first coronary VBT procedure was performed in Venezuela by Condado and coworkers in 1994. This feasibility study in 21 patients with 22 coronary lesions utilized a 192Ir source inserted by monorail into a closed-end lumen catheter after balloon angioplasty. The prescribed dose was 20 to 25 Gy to a distance of 1.5 mm from the center of the source, but the actual delivered dose to the luminal surface ranged from 19 to 92 Gy. The TLR rate was 18% at 2-year follow-up. The 5-year follow-up results confirmed the feasibility and durability of brachytherapy in reducing restenosis (9).
The Washington Radiation for In-Stent Restenosis Trial (WRIST) was initiated in 1997 and randomized 130 patients with in-stent restenosis of native coronary arteries or saphenous vein grafts to 192Ir brachytherapy or placebo (11,12). Fixed dosimetry was used to prescribe 15 Gy to 2.0 mm radius for lumen diameter less than 4 mm and 2.4 mm radius for lumen diameter greater than 4 mm. At 6 months’ follow-up, TLR was significantly improved by radiation therapy from 63% to 14% and angiographic restenosis rate from 58% to 19%.
The GAMMA I trial was the first industry-sponsored multi-institutional trial to determine the effectiveness of VBT for in-stent restenosis in native coronary arteries (13). The GAMMA I trial led to FDA approval of the Checkmate System by Cordis Corporation for in-stent restenosis of native coronary arteries. This study was a placebo-controlled, double-blind, randomized trial conducted at 12 institutions. The study randomized 252 patients to receive VBT or placebo. Dosimetry similar to the SCRIPPS-1 trial was utilized. The TLR rate was improved from 45% to 24% at 9 months and angiographic restenosis rate, from 50.5% to 21.6% at 6 months in favor of VBT over placebo. The late thrombosis rate in this study was 5.3%. Late thrombosis occurred most often in patients who had stents placed at the time of radiation therapy delivery. Although the results of this trial were not as robustly in favor of VBT as the SCRIPPS-1 trial, it was felt that this was associated with a learning curve for IVUS dosimetry in this multi-institutional trial.
The WRIST PLUS trial maintained 120 patients on clopidogrel therapy for 6 months after VBT. At 6 months’ follow-up, this study showed that extended antiplatelet therapy prevented the complication of late thrombosis that was seen in the GAMMA I study (14).
Patients who undergo CABG surgery with saphenous vein grafts have approximately a 40% rate of graft failure at 10 years. These patients often undergo stent procedures that also have a high rate of failure. Waksman et al reported the results of a multicenter, placebo-controlled, prospective study done in patients with evidence of in-stent restenosis of saphenous vein grafts. They enrolled 120 patients after successful revascularization to either treatment with manually loaded 192Ir or placebo. A closed-end noncentered delivery catheter was utilized and 5 mm of overlap with normal segment of vessel was employed at both ends of the lesion to ensure adequate margin. The dose ranged from 14 to 15 Gy for vessel diameters of 2.5 to 4.0 mm and 18 Gy for vessel diameter greater than 4.0 mm. There was a significant difference in the TLR rate in favor of the 192Ir arm versus placebo (17% vs 37%) at 12 months’ follow-up. Radiation therapy was the only significant predictor of freedom from a major cardiac event (MACE) at 12 months’ follow-up. The authors concluded that these data supported the use of γ-emitting radiation therapy for in-stent restenosis of saphenous vein grafts.
β-Emitting Sources
The START trial led to the FDA approval of the Beta-Cath system by Novoste Corporation for in-stent restenosis of native coronary arteries (15,16). This double-blind trial at more than 55 centers in the United States and Europe randomized 476 patients with in-stent restenosis to VBT or placebo. The prescribed dose at a reference distance of 2 mm from the source center was 18.4 Gy if the vessel diameter was between 2.70 and 3.35 mm and 23.0 Gy if the vessel diameter was between 3.36 and 4.0 mm. TLR rates were 26.8% versus 17% at 8 months’ follow-up in favor of the VBT arm over placebo. The 8 month angiographic restenosis rate (greater than 50% lumen diameter stenosis) within the area treated with radiation therapy was 45.2% versus 28.8% in favor of the VBT arm. In this study, the radiation sources were not successfully delivered in 0.6% of cases and the delivery catheter was not delivered in 1.3% of cases. The authors concluded that β-emitting VBT was both safe and effective in treating patients with in-stent restenosis.
The START 40/20 trial had the same enrollment criteria as the START trial, but the source train was 40 mm instead of 30 mm. This study found a lower geographic miss rate with the longer source train compared with the 30 mm source train used in the START trial (17).
The INHIBIT trial led to FDA approval of the Galileo system by Guidant Corporation for in-stent restenosis of native coronary arteries (18,19). This trial was carried out at 27 institutions and enrolled 332 patients. Three hundred fourteen of these patients successfully received the intended treatment. Lesions less than 47 mm were treated. This study used an automated delivery and retrieval system and a 32P β-emitting source. The catheter was centered and 20 Gy was prescribed at 1 mm from the catheter surface. Both the TLR and angiographic in-stent restenosis rates were significantly improved in the radiation therapy arm when compared with the placebo arm. The TLR rate was improved from 26% to 8% in favor of the VBT. The source was 27 mm in length and manual repositioning of the source was permitted for lesions that exceeded the source length. When repositioning manually, no overlap greater than 2 mm was permitted and no gap greater than 1 mm was permitted. No significant problems with the manual repositioning technique were identified in this trial.
In summary, for in-stent restenosis of native coronary arteries, the GAMMA I, START, and INHIBIT trials led to FDA approval of the Checkmate, BetaCath, and Galileo systems, respectively. The approval of VBT was unique in radiation oncology as the FDA-mandated level I evidence supported by multi-institutional, randomized trials prior to routine clinical use. Since 1995, more than 6,000 patients have enrolled in 50 protocols. VBT also presented the first-time multiple specialists including radiation oncologists, interventional cardiologists (ICs), and medical physicists were required to be part of the team delivering VBT.
De Novo Stenosis
VBT was not FDA approved for de novo coronary artery stenosis. The largest ever multi-institutional, double-blind randomized trial carried out for VBT was the BETACATH trial for coronary de novo stenosis (20). In this trial, 1,456 patients were randomized after balloon angioplasty to receive VBT or no treatment. If the angioplasty was not felt to be adequate, patients received stenting after VBT or placebo. There was no statistically significant difference in TLR between VBT and placebo. At 8 months’ follow-up, the angiographic in-lesion restenosis rate was improved by VBT versus placebo in patients who had angioplasty without stenting (34% vs 21%), but patients underwent edge failures that negated this benefit. Moreover, in the stented group, there was an increase in restenosis in the VBT arm versus placebo (35% vs 45%). There appeared to be hyperproliferation at the edges of the treated volume in the VBT group in patients who were stented. This study identified late thrombosis as the significant complication of VBT in stented patients. This has led to the use of long-term antiplatelet therapy to prevent late thrombosis in patients receiving VBT. Therefore, VBT has not been proved to be of benefit in patients with CAD who present with de novo stenosis. The geographic miss inherent in performing brachytherapy before the final injury length had been determined was the most likely cause for failure in this trial.
CURRENT PRACTICE
Since the advent of VBT, competing technologies have been developed, which include drug-coated stents, photodynamic therapy, sonotherapy, and gene therapies. Of these, DESs have largely transformed clinical care in interventional cardiology. The DES consists of three components: stent, drug, and a releasing mechanism. The drugs are immunosuppressive and antiproliferative agents. There are multiple approved DES in the United States, including the Cypher, Taxus, Endeavour, and Xience V stents. The Cypher stent utilizes the drug sirolimus, which is a weak antibiotic, but a powerful immunosuppressant. Sirolimus blocks cell cycles from progressing from G1 to S phase, preventing proliferation and migration of SMCs. The Endeavour and Xience V stents use zotarolimus and everolimus, respectively, which act similarly as sirolimus. The Taxus stent utilizes paclitaxel, which is an antineoplastic agent. Paclitaxel binds to tubulin, blocking microtubule disassembly, preventing the cell from moving from G2 to M phase. It interferes with the mitotic spindle apparatus, and hence blocks smooth muscle cell migration. It interferes with microtubule functions, affecting mitosis and extracellular secretion (21).
The first DES was approved by the FDA in April 2003. Several large, prospective, randomized trials have confirmed the efficacy of DESs as an antiproliferative treatment following revascularization with angioplasty. In the SIRIUS trial, 1,058 patients with de novo coronary stenosis undergoing PCI were randomized to sirolimus-eluting stent or bare stent. The sirolimus-eluting stent resulted in significant reduction in clinical restenosis compared with control, with only 8.9% of treated patients experiencing restenosis (22). In the Taxus-IV study, the polymer-based, paclitaxel-eluting Taxus stent had a 1-year rate of TLR of 4.4% compared with 15.1% for the identical-appearing bare-metal EXPRESS stent (23).
The advent of DES therapy and its success in preventing restenosis of de novo stenosis has mitigated the enthusiasm for VBT. The two published prospective randomized trials that compare drug-coated stents with brachytherapy for in-stent restenotic lesions are the SISR and TAXUS V trials. Both studies show superiority of DES over brachytherapy for in-stent restenosis within bare-metal stents. SISR is a prospective, multicenter, randomized trial of 384 patients with in-stent restenosis who were enrolled between February 2003 and July 2004 at 26 academic and community medical centers to receive either VBT (n = 125) or the sirolimus-eluting stent (n = 259) (24). The main end points measure target vessel failure (cardiac death, myocardial infarction, or TVR) at 9 months’ postprocedure. The rate of target vessel failure was 21.6% (27/125) with VBT and 12.4% (32/259) with the sirolimus-eluting stent (relative risk [RR]: 1.7; 95% confidence interval [CI]: [1.1–2.8]; P = .02). TLR was required in 19.2% (24/125) of the VBT group and 8.5% (22/259) of the sirolimus-eluting stent group (RR: 2.3; 95% CI: [1.3–3.9]; P = .004). At follow-up angiography, the rate of binary angiographic restenosis for the analysis segment was 29.5% (31/105) for the VBT group and 19.8% (45/227) for the sirolimus-eluting stent group (RR: 1.5; 95% CI: [1.0–2.2]; P = .07). Compared with the VBT group, MLD was larger in the sirolimus-eluting stent group at 6 month follow-up (mean [SD]: 1.52 [0.63] mm vs 1.80 [0.63] mm; P <.001), reflecting greater net lumen gain in the analysis segment (0.68 [0.60] vs 1.0 [0.61] mm; P < .001) due to stenting and no-edge restenosis. This study demonstrated that sirolimus-eluting stents result in superior clinical and angiographic outcomes compared with VBT for the treatment of restenosis within a bare-metal stent. The 3-year follow-up indicated the superiority of sirolimus-eluting stents over VBT, but the updated 5-year outcomes showed no significant difference in survival free from TLR, target vessel vascularization, or MACE (25,26).
The TAXUS V ISR trial is also a prospective, multicenter, randomized trial conducted between June 6, 2003, and July 16, 2004, at 37 North American academic and community-based institutions in 396 patients with in-stent restenosis of a previously implanted bare-metal coronary stent (vessel diameter: 2.5–3.75 mm; lesion length < 46 mm) (27). Patients were randomly assigned to undergo angioplasty followed by VBT with a β source (n = 201) or paclitaxel-eluting stent implantation (n = 195). Clinical and angiographic follow-up at 9 months were performed to assess end points. Diabetes mellitus was present in 139 patients (35.1%). Median RVD was 2.65 mm and median lesion length was 15.3 mm. In the VBT group, new stents were implanted in 22 patients (10.9%) and in the paclitaxel-eluting stent group, multiple stents were required in 57 patients (29.2%), with median stent length of 24 mm. Follow-up at 9 months was complete in 194 patients in the VBT group and 191 patients in the paclitaxel-eluting stent group (96.5% and 97.9%, respectively). For VBT and paclitaxel-eluting stents, respectively, the 9 month rates for ischemic TLR were 13.9% versus 6.3% (RR: 0.45; 95% CI: [0.24–0.86]; P = .01); for ischemic TVR, 17.5% versus 10.5% (RR: 0.60; 95% CI: [0.36–1.00]; P = .046); and for overall MACE, 20.1% versus 11.5% (RR: 0.57; 95% CI: [0.35–0.93]; P = .02). Similar rates of cardiac death or myocardial infarction (5.2% vs 3.7%; RR: 0.71; 95% CI: [0.28–1.83]; P = .48) and target vessel thrombosis (2.6% vs 1.6%; RR: 0.61; 95% CI: [0.15–2.50]; P = .72) were observed between the VBT and paclitaxel-eluting stent groups. Angiographic restenosis at 9 months was 31.2% with VBT and 14.5% with paclitaxel-eluting stents (RR: 0.47; 95% CI: [0.30–0.71]; P < .001). This study concluded that the treatment of bare-metal in-stent restenotic lesions with paclitaxel-eluting stents rather than angioplasty followed by VBT reduces clinical and angiographic restenosis at 9 months and improves event-free survival. The updated 2-year outcomes showed that ischemia-driven TVR was significantly better with paclitaxel-eluting stents compared to VBT (18.1% vs 27.5%, P = .03), but there was no significant difference with regard to death, myocardial infarction, or target vessel thrombosis (28).
CORONARY ARTERY VBT IN THE ERA OF A DES WORLD
The success of DES therapy in reducing rates of restenosis is a significant advance in the care of patients. Although the enthusiasm for VBT has been diminished by the success of DES therapy, there does remain a future for VBT in the care of patients. The results of the SISR and TAXUS V studies do not address groups of patients with in-stent restenosis (ISR) after DES placement. The 9 month failure rate after DES for ISR is 15% to 20% from these two randomized studies. Of note, Steinberg et al demonstrated that TVR was higher after DES implantation after in-stent restenosis from initial DES placement versus from initial bare-metal stent placement, which indicated a therapeutic challenge for ISR after initial DES placement (29).
Torguson et al presented data from the RESCUE Registry, a multicenter international electronic registry for patients who receive VBT for recurrent restenosis following DES (30). Sixty-one patients received VBT following DES and were compared with 50 patients who received additional DES (Taxus or Cypher stents) after DES restenosis. The patients’ demographic and angiographic characteristics were similar in both groups. VBT appeared to be safe. At 6 months, TVR rates were 3.3% versus 16% (P = .04) in favor of VBT. TLR rates were 3.3% versus 8% (P = .24) in favor of the VBT as well. At 8 months, VBT was associated with fewer MACEs compared with repeat DES, 9.8% versus 24%; P = .044.
At Scripps Clinic, the clinical experience on the first 41 patients treated with VBT following DES has been reviewed. Median follow-up is 8 months. TLR rates are 22% and TVR failure rates are 2%. Bonello and coworkers also demonstrated the effectiveness of VBT for DES ISR in a study of 99 consecutive patients who presented with ISR following DES implantation in 122 lesions. A high proportion of the patients presented with complex lesions, including diffuse or proliferate pattern of restenosis. At 12 month follow-up, the TLR was 11% and the overall MACE rate was 26%. Patients with multiple episodes of ISR to the same site had a TLR of 16% and MACE rate of 36% (31). Predictors of diffuse-type ISR following DES include the use of paclitaxel-eluting stents and smaller post-stenting minimal luminal diameter (32).
VBT has been demonstrated to be effective at treating complex ISR including diffuse ISR. Hang et al performed a study of 50 patients with diffuse ISR who underwent cutting balloon angioplasty followed by VBT with a liquid β-emitter rhenium-188 (188Rh)-filled balloon (33). With a mean follow-up duration of 6 years, the MACE rate was significantly reduced with VBT compared to control patients (20% vs 56%, P = .019). Six-month angiographic restenosis was noted in 16% of the VBT patients and 48% of the control patients (P = .025). However, Park and coworkers presented a study of 129 patients with diffuse ISR randomized to sirolimus-eluting stent versus VBT (34). Angiographic restenosis at 6 months was 8% versus 30% in favor of sirolimus stents. TLR was 4.6% versus 18.8% (P = .014) in favor of sirolimus-eluting stents. These are early results and, as noted from the SISR experience, a late catch-up phenomenon may still occur.
Although DES is an effective system for preventing vascular restenosis, given the number of DES procedures, even a small percentage of patients who fail to be successfully managed with the therapy could result in thousands of patients requiring VBT. Moreover, subsets of patients such as patients with diabetes and those with longer, more complex lesions are less successfully managed with DES therapy. These patients may be candidates for VBT.
PERIPHERAL VBT
Noncoronary peripheral atherosclerotic vascular disease (PVD) affects more than 10 million people in the United States and 20% of people older than 70 years. Up to half of these patients have symptomatic lower extremity PVD. The most common symptom of PVD is intermittent claudication, defined as pain on walking that is relieved by rest. In PVD, the femoral and/or popliteal arteries tend to be highly diffuse, long chronic total occlusions or long segments with multiple narrowed areas. Treatment options for PVD include conservative medical management and more aggressive invasive therapies including PTA, surgical bypass, and amputation. An estimated 600,000 interventional procedures are performed for the treatment of lower limb arterial disease each year, including PTA, bypass surgery, and amputation.
Patients with carotid artery disease have significant morbidity and mortality rates. Approximately 5% of the hypertensive patients have renal artery stenosis. Approximately 400,000 peripheral angioplasties are performed in the United States annually. Unfortunately, just as in the case of coronary arteries, restenosis is a significant problem in PVD.
Compared with open bypass surgery, nonsurgical interventions offer the potential for decreased operative mortality, shorter hospital stays, and fewer wound complications. These advantages can translate into increased cost-effectiveness if patency rates equal those of a vein bypass. However, the need for repeat procedures can be the “Achilles heel” of these nonsurgical interventions. The initial benefits of lower cost of nonsurgical interventions as compared with open bypass can be offset by repeat procedures needed to maintain patency. Therefore, the indication for nonsurgical intervention as opposed to surgery or conservative medical management still remains the matter of debate if one considers the cost factor.
Parallel to the effort for the coronary artery, investigators have attempted to expand the idea to utilize radiation to reduce the risk of restenosis in PVD because the basic biology of restenosis is found to be similar. However, there are some major differences in clinical, anatomic, and technical aspects of the two areas (35).
1. PVD involves more organs than CAD. Therefore, there are more diverse clinical situations, manifestations, and end points. In addition to the typical vessel parameters such as late loss or late loss index, it is challenging to define measurable clinical end points for a diverse group of “host” organs (extremities, kidney, liver, etc), which are different not only in anatomy but also in complex functions.
2. Anatomy. There are differences in vessel dimensions such as length, diameter, thickness, curvature, and orientation. Unlike coronary vessels, most peripheral vessels have a diameter greater than 3 mm and, in fact, are typically approximately 7 to 10 mm; some may be as large as 20 mm (eg, the aorta). The peripheral arterial tree, because it is larger and more diverse than the coronary circulation, poses very different clinical scenarios and end points.
4. Technical aspects. Owing to a larger vessel diameter, endovascular brachytherapy using seed- or wire-based catheter system requires high-energy γ source (192Ir) because β or low-energy γ sources would underdose the subintimal walls. Most trials in peripheral vascular systems currently utilize a high dose rate (HDR) 192Ir source, because the manual loading technique with low dose rate seeds typically used in the coronary system requires higher activity (more exposure to personnel) and longer treatment time; these pitfalls are rectified by the HDR remote afterloader system. However, the manual afterloader system has two advantages over the HDR remote afterloader system: (a) source positioning can be adjusted in vivo and (b) angulation of the delivery catheter can be recognized by the operator of the manual afterloader and can then be corrected by a more forceful push on the source wire and change in the torque as need be. In contrast, the HDR remote afterloader may abort the treatment. Although it is possible to deliver HDR treatment with sources other than high-energy γ emitters in the vascular lab with modification of room to meet shielding requirements, almost all treatments with high-energy γ sources such as 192Ir are delivered in the department of radiation oncology. Therefore, the logistics of patient transportation must be considered in protocol design: increase in overall catheterization time, minimal movement of catheter systems, anticoagulation, and personnel are some mitigating factors. Furthermore, the use of a centering device may be more critical for peripheral vascular system than for coronary vessels to assure dose uniformity.
5. The impact of the DES is not as much in PVD as in the coronary system. The SIROCCO study, which used sirolimus-eluting nitinol stent, did not find the impact of DES over an uncoated stent. The in-stent mean percent diameter stenosis was 22.6% in the DES group versus 30.0% in the uncoated stent group (P = .294) at 6 months. DES had late recurrence and mechanical problems (36).
Superficial Femoral and Popliteal Arteries
These vessels are the most affected vessels in the body with more than 100,000 interventions per year. There is often poor distal runoff, which creates a high resistance and low flow state. Because they are muscular (distributing) arteries, the superficial femoral, popliteal, and tibial vessels have high rates of restenosis after percutaneous interventions. The primary patency rates for PTA of femoropopliteal lesions average 61% at 1 year (47%–86%). At 3 to 5 years, the patency rate after endovascular treatment ranges 38% to 58% (37). Three-year patency rates range from 61% for claudicants with stenoses to 30% in patients with critical limb ischemia and occlusions (38). Femoropopliteal lesions that have been stented have 3-year patency rates of 58% to 66% (39). Beside the coronary vessels, these vessels accumulated the most clinical studies in evaluating the role of VBT in reducing restenosis.
Tables 14.2 and 14.3 list the important studies, of which an excellent review was done by Schillinger and Minar (40). Almost all studies were performed in Europe except for the Peripheral Artery Radiation Investigational Study (PARIS) that was done in the United States and Europe. All studies utilized remote afterloading HDR 192Ir sources. Unlike the studies in coronary vessels, these studies examined the use of VBT for prophylaxis of restenosis after PTA of de novo lesions, prophylaxis of restenosis after PTA of recurrent lesions, and prophylaxis of in-stent restenosis. Studies were done with and without additional bare metallic stent after VBT.
The Frankfurt trial reported by Böttcher et al was the first to present the safety and feasibility of VBT in PVD (41). Twenty-eight patients with post-PTA recurrent lesions (mean length = 6.7 cm) in the superficial femoral artery (SFA) were treated with repeated PTA plus stent implantation and VBT. A dose of 12 Gy was given at a fixed radius of 3 mm using HDR 192Ir and a noncentering 5-F catheter. The patency rate at 5 years is 82%. Three patients developed restenosis and two presented with thrombotic occlusion at 16 and 37 months.
The Switzerland trial reported by Zehnder et al (42) on 100 patients with recurrent SFA lesions after PTA who were randomized to repeated PTA alone versus PTA plus VBT (12 Gy at 3 mm radius using a noncentering 5-F catheter and HDR 192Ir). At 1 year, the rate of restenosis was lower in the VBT group at 23% versus 42% for the control group (P = .028). Gallino et al (47) reported another Switzerland multicenter trial with 335 patients who were prospectively randomized to a 2 by 2 factorial design with PTA alone, PTA plus VBT, PTA plus probucol, and PTA plus VBT and probucol. A high rate of late thrombotic reocclusion (27%) in patients receiving stenting and VBT was noted. No late thrombotic reocclusion was seen in patients receiving PTA and VBT. Despite this, the 6 month restenosis rate is still lower in patients receiving VBT (17% vs 35%, P < .01), and probucol made no difference.
Table 14.2 Peripheral artery radiation therapy (part) trials
Table 14.3 Peripheral artery radiation therapy (part) trials on de novo lesions
The PARIS study is the only FDA-approved study at 12 centers in the United States and Europe. In the initial phase II, VBT was delivered successfully to 35 of 40 patients at four centers using a specially designed double lumen–centering catheter. The angiographic restenosis at 6 months was 17% and the clinical restenosis at 12 months was 13%. The second phase of this study was a prospective randomized, double-blind control study with 300 patients at 12 centers in the United States and Europe. Only 203 patients (of the 300 intended) were enrolled. A dose of 14 Gy was prescribed to the adventitia, which was defined at 2 mm depth from the surface of the balloon. The preliminary data were presented by Waksman (49). Unfortunately, angiographic follow-up data were available only in 75 of the 203 patients (37%). Binary restenosis rates were 28.6% in the VBT compared with 27.5% for the control group (P = .92). There were no differences in percent stenosis, TVR, late loss, ankle-brachial index (ABI), or maximum walking distance. There is no further follow-up publication of this study. For patients more than 65, maximum walking times at 6 and 12 months were better in the VBT group. There are several criticisms of the results. First, the enrollment was poor: Only 203 patients of the intended 300 enrolled. Second, the follow-up angiography was available only for 75 patients. Third, the restenosis in the control arm was lower than expected rates of 40% to 60%. This study should be considered incomplete rather than negative.
Other Peripheral Vascular Systems
The infrapopliteal vascular system is smaller than the femoral–popliteal system. It is more difficult to manage and responds less to conventional treatment. Early results of percutaneous revascularization for occlusive disease below the knee were disappointing. The risk of complications and restenosis remains increased in the smaller and often more diffusely diseased and calcified vessels. Brown et al from St Luke’s/Roosevelt Hospital Center performed PTA in 11 patients facing reconstructive surgery for limb salvage. Of the 16 diseased tibial runoff vessels, 15 were successfully dilated. They reported the restenosis rate at 44% at 2 years after PTA (56). Jahnke et al from Germany reported their experiences during an 18 month period, when a total of 19 infrapopliteal lesions in 15 consecutive patients were treated primarily by high-speed rotational atherectomy (HSRA) by using the Rotablator device. Control angiography was carried out at 6 months in 9 of 15 patients, allowing direct assessment of 12 of 19 treated lesions. Among six high-grade restenoses and five total occlusions in the treated vascular segments, only one arterial lumen (of 12) remained patent without presenting a hemodynamically relevant restenosis (57).
As for the renal vascular system, renal occlusive disease is the major cause of hypertension and renal failure. Estimates of the prevalence of renovascular hypertension vary from less than 1% in the general population to 30% to 40% at some referral centers. Atherosclerotic renovascular disease is the cause of nearly 75% of renal stenoses (58). Fibrous dysplasia (a group of congenital dysplasias of various layers of the arterial wall) accounts for most of the remaining cases of renovascular disease. In 1978, Grüntzig et al reported the first successful treatment of renal artery stenosis with a balloon catheter (59). PTA has been shown to be very effective for renal artery stenosis due to medial fibroplasia. Most series have shown rates of 90% or higher for technical success and clinical benefit. Long-term patency, including secondary patency after redilatation, reportedly exceeds 95%. However, results of balloon angioplasty for treatment of atherosclerotic renal artery stenoses have been disappointing, especially in the ostial location. The alternative to balloon angioplasty for percutaneous revascularization is stent placement in the renal artery. Reports of several studies involving metallic stents to treat renal artery stenosis have been published, including one study that focused specifically on ostial lesions resistant to balloon dilatation (60). The results are encouraging. Technical success rates of almost 100% and long-term secondary patency rates of higher than 90% after repeated intervention have been reported. Rates of restenosis after initial intervention have ranged from 10% to 20%. Clinical cure occurs in approximately 20% of cases of stenosis of atherosclerotic origin and approximately 60% of lesions resulting from medial fibroplasia. In addition, clinical success (defined as cure of or improvement in hypertension) in lesions of either origin ranges from 80% to 90%. Percutaneous revascularization is also performed to preserve renal function in patients with greater than 75% stenosis either bilaterally or unilaterally when only one kidney is present. In appropriately selected patients, improvement in or stabilization of renal function can be expected in 80% to 90% of cases.
The treatment of choice for renal artery stenosis is generally percutaneous angioplasty. Most lesions require stenting because of the aorto-ostial location of atherosclerotic renal artery stenosis. Many studies have shown that the rate of restenosis after PTA ranges from 45% to 65% and that after PTA and stenting of renal arteries the rate is between 15% and 25%. In the GREAT trial, the restenosis rate in the bare-metal stent arm was 14.3% (61). The risk factors for a higher restenosis rate are smokers, vessels less than 4 mm in diameter, and longer follow-up duration. Unlike coronary arteries in which most restenosis occurs in the first 6 months after intervention, there is a late recurrence rate with renal system (62). The Washington Hospital Center used HDR 192Ir to treat 10 patients before PTA, and they reported a patency rate of 90% at 1 year (63). In another publication from Switzerland, Stoeteknuel-Friedli et al reported treating 11 patients with ISR with HDR 192Ir. They used the dose of 14 Gy at 5 mm radius, and reported the patency in eight of the 11 patients at 18 months (64). Another report from the University of Kentucky by Jahraus et al is on five patients with ISR treated with 90Y/90Sr source. They reported the patency in four of the five patients at 7 months (65).
In the United States, approximately 300,000 patients suffer from end-stage renal disease (ESRD); 85% of these patients will be treated by hemodialysis. In the absence of a kidney transplant, these patients will require artificial hemodialysis for their lifetime. Patients undergoing hemodialysis require a direct artery–vein access that is easy to locate and that will provide optimal blood flow during treatment. Unfortunately, a number of hemodialysis patients do not have adequate vessels to form a fistula (a connection) between a vein and an artery; therefore, a synthetic graft must be inserted. Synthetic grafts are the most widely used type of vascular access in the United States, the most common being the polytetrafluoroethylene (PTFE) graft. Preservation of access sites is of paramount importance as patients are being treated with hemodialysis for longer periods (almost 50% are treated for 5 years). Balloon angioplasty has been used for maintaining, rather than restoring, the graft when tissue growth narrows the passageway. Although surgery is reasonably effective, there are associated problems. Balloon angioplasty is usually required over a long term, because uncontrolled tissue growth within the graft is very common. The long-term follow-up information on PTFE grafts shows that 60% have narrowed within the first year and 40% have narrowed by 2 years. Venous stenosis and thrombosis (due to venous neointimal hyperplasia) are the most common cause of hemodialysis vascular access dysfunction with a 50%, 1-year primary patency for new grafts and a dismal 40% 3 month survival for thrombosed grafts (66). However, despite the magnitude of the clinical problem, there are currently no effective therapies for this condition. Two modalities of radiation have been used to treat the AV graft: external beam from linear accelerator and brachytherapy.
In 1994, a phase I to II study at Emory University was done to treat patients who had failed PTA of arteriovenous dialysis grafts using the HDR 192Ir. Waksman et al reported a 40% patency rate at 44 weeks; however, the long-term results of this study were similar to PTA without radiation (67). Parikh and Nori et al reported a phase I to II study utilizing external radiation doses of 12 and 18 Gy for AV dialysis shunts in 10 patients. The result is similar to the Waksman’s study. At 6 months, TLR was 40%, but at 18 months, all grafts failed and required intervention (68). Cohen et al randomized 31 patients to PTA or stent placement alone, followed by external beam radiation of 14 Gy in two fractions, and they reported restenosis rates of 45% versus 67% in the irradiated and control groups, respectively, at 6 months (69).
New studies are currently underway using low-dose external radiation to reduce restenosis of vascular access for AV grafts in hemodialysis patients, as are other studies using a centering device to deliver an accurate homogeneous dose of radiation after PTA. Beta Radiation for Treatment of Arterial–Venous Graft Outflow (BRAVO) was a pilot study utilizing the Corona system with a 90Sr/90Y β emitter. In a study of 10 patients with an average of 3.9 previous angioplasties to their AV graft, there was 60% primary patency and 80% cumulative patency at 12 month mean follow-up (70). However, the follow-up randomized BRAVO II trial failed to show a benefit of endovascular radiation therapy, though this was partly due to low study enrollment due to business and recruitment issues (71).
As for the iliac vascular system, the iliac arteries are conductance vessels (elastic) with a high elastin content in their media. Consequently, the rate of restenosis is expected to be relatively low. The 1-year patency rate after PTA of iliac stenoses averages at 78% (67%–92%), whereas for iliac occlusions, it averages at 68% (59%–94%). The 1-year patency rate for stenting of iliac stenoses averages at 90% (78%–97%), and for iliac occlusions it averages at 72% (68%–94%) (72). In the Dutch Iliac Stent Trial, clinical success was similar at 2 years for claudicants who underwent PTA or stenting of iliac disease (73). The angiographic restenosis rate after stenting in the Palmaz Multicenter Registry was 8% at 9 months, and in the Wallstent Registry, it was 12% at 6 months. In the more recent CRISP trial, the 12 month primary patency rate for the SMART stent was 94.7% (as determined by duplex and ABI).
As for the hepatic vascular system, portal hypertension can be treated by a transjugular intrahepatic portosystemic shunt (TIPS), which creates an intrahepatic tract between the hepatic and portal veins using an angiographic technique through a transjugular approach. This tract is then dilated and kept patent by placement of a metallic, self-expanding, compliant stent. Shunt stenosis can be seen in up to 50% of patients within 6 months. The development of TIPS malfunction is multifactorial. Three causes are (a) acute thrombosis (less than 5% incidence); (b) stent retraction (2% incidence); and (c) most commonly, shunt stenosis. In Sanyal’s review (74) of the natural history of TIPS, 51 of the 70 patients (73%) developed shunt stenosis within 6 months of TIPS placement. Three patterns of stent stenosis are observed: (a) hepatic vein stenosis, (b) diffuse neointimal hyperplasia, and (c) both factors combined. The three patterns of stent stenosis have been amenable to balloon dilatation and placement of another stent within the original stent. However, the repeated interventions required to correct failure of the initial shunt add significant risk to the patient, as well as increase health care cost. It was hypothesized that minimizing the process of neointimal hyperplasia at the time of initial TIPS placement would reduce the risk of restenosis, and therefore the probability of TIPS failure.
So far, VBT experience for the hepatic vascular system has been limited. Pokrajac et al (75) reported a study from the University of Vienna with five patients with in-stent restenosis of TIPS. Redilatation was done in all five patients 6 months after first stenting because of restenosis (greater than 50% stent lumen reduction) or occlusion of the stent. The authors reported that normal patency (less than 50% lumen reduction) of the stent was achieved at 44 months’ follow-up (based on duplex ultrasound and portography) in all three patients with liver cirrhosis, whereas further revisions were necessary in the two patients with Budd–Chiari syndrome (after 5.5 and 18 months). No acute, subacute, or late side effects of radiation were seen. A phase I to II study was initiated at Scripps Clinic using a centering catheter (PARIS catheter) and IVUS-guided dose prescription. The distance from the center of the catheter to the transhepatic tract (the “source-to-target distance”) was measured by IVUS. A dose of 10 Gy was prescribed to a radial distance equal to the farthest source-to-target distance plus an additional 2 mm. The dose to the shortest source-to-target distance was limited to 30 Gy. Because the centering balloon had a minimum radius of 4 mm, it was expected that the maximum dose to the closest tract wall would be less than 30 Gy. The minimum dose delivered to the target region will determine the control rates; the maximum dose delivered to the sensitive tissue will determine the complication rate. Treatment length was equal to the stent length plus 1.5 cm proximally and 1.5 cm distally. Figure 14.3 shows the localization and treatment planning radiograph of one of the few TIPS brachytherapy cases performed with the PARIS catheter in the United States.
As for the carotid vascular system, carotid artery disease accounts for approximately one third of strokes. Carotid endarterectomy, the accepted conventional therapy for obstructive carotid disease, has been shown to be superior to medical therapy for both symptomatic and asymptomatic obstructions (76). However, there is some interest in percutaneous treatment as an alternative to surgical endarterectomy. Carotid artery PTA and stenting are rapidly emerging as an efficacious modality for treating symptomatic and asymptomatic carotid artery occlusive disease. A recent review by Wholey et al (77) summarized the worldwide experience of carotid artery stent placement from nonrandomized, observational studies. The reported rates of major and minor strokes and death appear to be similar to those for endarterectomy involving high-risk patients. As an elastic (conductance) artery, the restenosis rate is expected to be low. Indeed, the restenosis rate has ranged from 5% to 8% after carotid PTA and/or stenting (78). Risk factors identified for restenosis after carotid artery stenting include female gender, advancing age (in contrast to carotid endarterectomy, in which younger age predicts restenosis), and, variably, the number of stents implanted. Restenosis lesions after carotid endarterectomy are at higher risk of in-stent restenosis. Interestingly, residual stenosis after the carotid artery stenting procedure, but not vessel size after the procedure per se, has been found by some to be a predictor of restenosis (79). The other risk factor is irradiation from previous head and neck malignancy. There are animal data supporting the use of radiation in reducing restenosis in rabbits and rats. Investigators from Germany (80) reported the use of VBT in 68 male New Zealand White rabbits after endothelial denudation of the right common carotid artery with a Fogarty catheter. Endovascular irradiation was performed with a rhenium-188 (188Re)-filled 3.0 mm balloon catheter using different dosages (0, 7.5, 15, 30, 45, and 60 Gy at the surface of the vessel). Then 4 weeks after the intervention, the vessels were excised and histologically analyzed. They found that at 7.5 Gy, the intimal area did not differ significantly from the control, neointimal hyperplasia was decreased significantly at 15 and 30 Gy and completely inhibited at the highest dosages of 45 and 60 Gy. There are several case reports of success of VBT in this setting in human data (81–83). There is some consideration in the use of VBT in reducing risk of restenosis after carotid stenting (84).
Figure 14.3 Anterioposterior localization and treatment planning radiograph of one of the few transjugular intrahepatic portosystemic shunt (TIPS) brachytherapy cases treated on compassionate use exemption in the United States. Note that a previous nonfunctioning TIPS stent is to the side of the target stent. Weak contrast is used to show the balloon without undue attenuation of therapeutic dose. The treatment plan is a straight-line optimization to a given radius and length prescribed by the treating physician.
INDICATIONS FOR VBT
VBT has shown to be efficacious for various scenarios of restenosis in coronary arteries. However, due to the effectiveness of DES, routine use of VBT is uncommon. Compared with trials for CAD, PVD VBT trials have shown mixed results and remain an area of potential opportunity for further study. Nonetheless, in select cases, VBT remains useful. Potential candidates for VBT are as follows:
Patient with in-stent restenosis of native coronary arteries
Patients with in-stent restenosis of saphenous vein graft
VBT TEAM ROLES AND RESPONSIBILITIES
The applicator and source insertion for VBT are described in detail by the American College of Radiology (ACR) policy statement on the performance of coronary VBT (85). The procedure requires the presence of an IC, radiation oncologist (who is the authorized user of the delivery system), and medical physicist. The delivery of brachytherapy for coronary vessels requires coordination of the IC, radiation oncologists, and physicists to assure safe and proper treatment delivery with efficient utilization of time. This is also a requirement from the FDA. Additionally, prior to starting a VBT program, a quality assurance and improvement program should be in place involving the IC, radiation oncologist, and medical physicist. The roles and responsibilities of these specialists are defined.
Prior to VBT, the IC performs a preprocedure evaluation and assesses the patient’s status, including risk factors and prior interventions. The radiation oncologist performs a similar preprocedure evaluation of the patient and discusses with the IC the advisability of using VBT. The radiation oncologist should review the anatomic location of the diseased vessel(s) amenable to intervention, length of VBT intervention, angioplasty, stenting, and RVD with the IC. The radiation oncologist should determine the TVs together with the IC. Once VBT is deemed appropriate, the medical physicist makes appropriate preparations and orders the necessary radiation sources under the direction of the radiation oncologist. The physicist will calibrate the sources on arrival and ensure safekeeping of radiation sources. The physicist will also prepare sources for clinical use.
Informed consent for coronary intervention is obtained by the cardiologist and for VBT is obtained by the radiation oncologist. The consent should be obtained after a discussion with patient has covered the indications, the benefits, the risks, and alternative procedures as well as an explanation of the procedure itself in language that can be easily understood by the patient.
As for the actual VBT procedure, vascular access for coronary intervention is gained by the IC. The right femoral artery is the most common site for vascular access, and other sites include the left femoral artery bilateral radial, brachial, and axillary arteries. A guidewire is inserted at the access site over which a dilator is placed. An intravascular Teflon sheath is inserted over the dilator to secure the vascular access site. This sheath allows for rapid exchange of catheters, limits blood loss, and can be used to monitor the vascular pressures of the patient.
The common terminology for the diameter of a guiding catheter is French (F), and 1 F equals 0.33 mm. The size of interventional catheters has been rapidly decreasing and most interventional procedures can now be performed with 5 to 6 F catheters. Coronary guidewires are steerable and can be advanced through the guiding catheter into the coronary artery. The guidewire is used to advance the balloon catheter. The balloon catheter is made up of a support shaft to allow for advancement through the arterial lumen, a central lumen for the guidewire, and an inflation channel.
During the angioplasty procedure, the IC advances the balloon catheter into the area of stenosis and expands the balloon, with the intention of opening the stenosis. Stenting the previously narrowed region became an extremely popular and effective means to prevent restenosis after angioplasty. Next, the radiation oncologist is typically called to the cardiac catheterization lab after the coronary intervention has been initiated by the IC. The medical physicist will survey the catheterization laboratory and make appropriate preparations. The physicist will also survey the patient before, during, and after the VBT procedure. Once the target vessel(s) is identified, the TV is determined jointly on the basis of the lesion size, injured length of vessel, and vessel diameter. The delivered dose is determined on the basis of the diameter of the vessel and the current activity of the radioactive source, and is recorded in the prescription/written directive. The medical physicist will calculate treatment delivery times.
The IC is responsible for the general medical care of the patient while the patient is in the catheter lab. The IC performs the angioplasty and all physical manipulation of catheters within the coronary arteries. The radiation oncologist positions the source within the treatment catheter after consultation with the cardiologist regarding the location of the lesion and geometry of the vascular anatomy. The IC then checks the positioning of the source to confirm proper positioning. Documentation of position is usually by cineangiography, which is stored electronically. Both the IC and radiation oncologist monitor the entire delivery of radiation therapy. The radiation oncologist removes the source from the patient and oversees the surveying of the patient and room by the medical physicist at the close of radiation delivery. The IC then performs final imaging and recovers the patient. In case of radiation or medical mishap, the medical physicist will inform the appropriate regulatory authorities. Moreover, the ACR recommends that preestablished procedure guidelines for brachytherapy developed by the team of radiation oncologist, medical physicist, and IC be in place before performing VBT.
CORONARY BRACHYTHERAPY: DELIVERY SYSTEM AND TREATMENT PLANNING
The three delivery systems that have been approved by the FDA for coronary brachytherapy application include the Checkmate system by Cordis Corporation, the Beta-Cath system by Novoste Corporation, and the Galileo system by Guidant Corporation. Table 14.4 compares some physical properties of the three coronary treatment devices.
Although there are three FDA-approved systems, the Beta-Cath system by Novoste Corporation remains the only commercial coronary vascular device on the market (Figures 14.4 and 14.5). The Beta-Cath system, which is a β-emitting noncentering delivery system, contains a handheld manual device that uses a closed-loop hydraulic system with sterile water to deliver and retract a 30, 40, or 60 mm 90Sr/yttrium-90 (90Y) seed train to the target. The Beta-Cath system consists of four main components: the source train, transfer device, delivery catheter, and accessories. The Beta-Cath system contains sources that are 2.5 mm in length and 0.38 mm in diameter confined within a flexible steel coil between radiopaque end plug markers. The nonradioactive end plug markers are located proximally and distally to the radioactive sources in the jacketed train. The markers permit fluoroscopic verification of the source train arriving at the end of the delivery catheter for treatment. The advantage of the Beta-Cath system is the relatively short treatment times (3–5 minutes) and the absence of radiation exposure to catheterization laboratory staff. The long half-life of the isotope permits a total shelf life of 12 months. A potential disadvantage of this system is the inferior depth–dose gradient compared with the γ source, attenuation by calcifications or stents, and the lack of utility in larger vessels.
For lesions longer than the available source train, a “pullback technique” is used in which the most distal portion of the lesion is treated first, then the catheter is carefully pulled back to treat the more proximal lesion. At Scripps Clinic, the 60 mm source train is available and can be used with a pullback technique to cover a distance of 120 mm. This technique could also be used for treatment of lesions at vessel bifurcations. Significant overlap or gaps between the treatment fields should be avoided.
The GTV is the stenotic area itself. The CTV is the dilated part of the vessel. The PTV includes at least 5 mm proximal and distal to the CTV for the 30 mm source train and at least 10 mm for the 40 and 60 mm source trains (86,87). A dose of 18.4 Gy is recommended at 2 mm radius from the centerline of the source train axis for vessels with a reference diameter between 2.7 and 3.35 mm. For reference diameters between 3.35 and 4 mm and for most saphenous vein grafts, a dose of 23 Gy is recommended.
γ Versus β Sources
There exists a debate on whether γ– or β-emitting sources are better for VBT. A study comparing the dosimetry of β versus γ radiation sources for catheter-based VBT found that the dose reduction beyond a calcified plaque or a metallic stent could be greater than 20% for the β-emitting 90Sr, but was negligible for the γ-emitting 192Ir (88).
The advantages of γ-emitting sources include the fact that they have an improved depth–dose gradient compared with β-emitting sources and are not attenuated by calcium inside vessel lumens or by metallic stents. However, γ-emitting sources expose the catheter lab staff to increased ionizing radiation and require lead shielding to be placed in the catheter lab. The treatment times are longer with γ-emitting sources. On the other hand, β-emitting sources have shorter penetration than γ sources and can be attenuated by calcium or stents, leading to potential underdosing of the target. β-emitting sources may have longer half-lives, higher specific activity, and higher dose rate, and treatment times are shorter than with γ-emitting sources (89). The Beta versus Gamma Utrecht Trial (BEGUT) compared β– versus γ-emitting sources for use in VBT in a single-institution randomized, prospective study (90). The BEGUT was designed to compare safety and feasibility. The results of the study were negative with 3-year target vessel failure 45% for β– and 30% for γ-emitting sources, but was not statistically significant. Hence, the ideal isotope is that which is safest for the patient, operator, and public, and most efficacious. It is not possible to interchange isotopes between different systems, and so it is more appropriate to compare the results of systems rather than isotopes.
Table 14.4 Comparison of the physical properties of the three coronary treatment devices
Figure 14.4 Novoste Beta-Cath system is a handheld device with 90Sr seeds within Lucite shielding. The seeds are propelled with a closed hydraulic system by the radiation oncologist. The β sources pose less shielding issues.
Figure 14.5 Novoste Beta-Cath Catheter.
Figure 14.6 This is the most typical image used in interventional cardiology—two-dimensional representation of three-dimensional reality. Considerable skill and experience help the cardiologist choose the best plane of image to guide diagnosis and intervention.
Figure 14.7 A schematic of the orientation of the intravascular ultrasound (IVUS) fan of sound that generates the image. The images are real time, and still photos are selected to demonstrate structures and to take highly accurate measurements with the software.
Imaging Modalities
Two imaging modalities are used in VBT: angiography and IVUS. Images from angiography are obtained from fluoroscopy while contrast is given. The images are actually the shadow of the lumen of vessel, visualized by the contrast material. The images do not give any information about the wall of the vessels. These images are two dimensional or planar. The plane of the view is chosen by the cardiologist to best view the vessel of interest. Owing to the nature of the fluoroscopic imaging, the magnification factor is not the same at different parts of the image. The images can be static or in dynamic mode (cine). The images are stored for review. Despite all these downsides, this is the main modality used by the cardiologist during the intervention process. It allows the visualization of the metallic stent, guidewire, and other markers (Figure 14.6).
IVUS is a three-dimensional imaging modality. A high-frequency sound wave is sent radially to the vessel wall and its echo is received and processed electronically. Each axial IVUS image shows details of the lumen and the vessel wall. IVUS images are acquired like CT scan images; there is an automatic acquisition of images as the device is withdrawn along the vessel. These axial images can be combined and reconstructed to show the sagittal and coronal images. The measurement from these images is less subject to distortion so it is more accurate than angiography. There is a reference millimeter marker on the vertical and horizontal axes of the IVUS images. Most IVUS software allows some quantification on the image, such as distance, area, and volume measurement. Figure 14.7 shows a schematic of the orientation of the US waves. Figure 14.8 demonstrates clinical utility in coronary interventions.
Treatment Planning Issues
It is essential to treat the stenotic lesion with adequate margins to prevent edge restenosis. Edge restenosis is the term for higher-than-expected restenosis rates at the edges of the treated volume. Possible etiologies for the edge effect include geographic miss of the lesion, barotrauma secondary to balloon inflation distal to the treated volume, source movement during the treatment, and dose falloff and penumbra at the edge of the treated volume.
Figure 14.8 Three frames demonstrating intravascular ultrasound (IVUS) imaging. (A) Shows the stent as a white-dotted line most clearly seen superiorly. (B) Shows the IVUS catheter and the guidewire. (C) Demonstrates the lumen, the stent, and the external elastic membrane (EEM).
Source Movement Effect
Giap et al retrospectively reviewed source displacement during the cardiac cycle in 30 patients who underwent VBT at Scripps Clinic (91). They found a mean longitudinal source displacement of 1.1 mm (range: 0.0–5.4 mm). The authors recommend adding the contribution from source movement to the TV to ensure adequate coverage and avoidance of a geographic miss.
Figure 14.9 shows an example of longitudinal seed displacement during cardiac cycle. The two frames (A and B) were captured during the cineangiogram and they represent the maximal distal source displacement. If the vessel branch is used as the reference point, A shows only one seed distal to this reference point. B, which was captured less than 1 second later, shows two seeds distal to the reference point. After performing precise measurement and demagnification, the magnitude of the seed movement for the distal end is 3.3 mm. Similar calculations are done for the proximal end, and the value is 1.5 mm.
Barotrauma Effect
After angioplasty, a metallic stent is usually placed into the vessel by balloon expansion. Barotrauma refers to the injury to the vessel wall due to the balloon inflation or from the stent deployment balloon. The length of the balloon used for stent deployment is typically longer than the stent itself. Figure 14.10 shows a bare-metal stent on its deployment balloon. Distal and proximal regions of dilatation and barotrauma without stenting to protect from recoil are at risk for restenosis.
In a study from Scripps Clinic, current stent designs were reviewed, providing an assessment of barotrauma due to stent deployment in VBT, the barotrauma length averaged 1.7 mm (range: 0.5–2.5 mm) (92). Table 14.5 reviews nine commonly used stents and their corresponding barotrauma lengths. To minimize a marginal failure at the edges of the treatment volume, it is important to include the entire region of barotrauma in the treated length.
To Center or Not Center
Figure 14.11 shows the cross-sectional view of the three catheters in the treated lumen for the Cordis Checkmate, Novoste BetaCath, and Guidant Galileo systems, respectively. The Checkmate catheter is a noncentering single lumen; the BetaCath catheter is also noncentering but has a triple lumen hydraulic system; and the Galileo catheter is a centering and single-lumen system.
There was significant debate regarding whether centering the radiation source within the delivery catheter system would improve the safety and efficacy of VBT. Proponents of centering argued that regardless of the isotope used, centering the radiation source improves precision and reproducibility over noncentered sources. These proponents of centering argued that using a noncentered source can introduce significant variability in the dose received by the circumferential target (93). Others believed that centering is not important for coronary VBT (94). They argued that positive results in both animal and human trials had been observed without the use of centered delivery catheters and no study had shown a benefit to centering against noncentering. They also stated that variability of the lumen diameter and movement of the delivery catheter within the lumen leaves little capacity for reproducible centering within the coronary vasculature. It is important to note that it is logical to see the benefit of centering of the source within the treated vessel wall so that the radiation is distributed uniformly to the vessel wall. By using the centering catheter within a diseased lumen, which is often noncentric due to plaque, the source could be noncentered with respect to the vessel wall. Because the range of γ source is longer, the effect of noncentering was less significant for γ sources compared with β sources.
Figure 14.9 (A and B) Frame capture pictures from a cineangiograph demonstrating significant beat-to-beat movement of the treatment catheter during therapy.
Source: Courtesy Huan Giap, MD, PhD.
Figure 14.10 A typical stent deployed on its balloon. Note the tapering proximal and distal ends, contributing to barotrauma injury outside the stented region.
Edge Failure (“Geographic Miss”)
When restenosis occurs after VBT, the renarrowing is found at the treatment edges in one third to one half of the patients. The etiology of edge failure is likely to be multifactorial, but most likely results from inadequate radiation dose delivered to injured lesion margins. Many factors can lead to higher-than-expected edge failure. These include geographic miss, which arises from misalignment of the radioactive source within the injured segment of the vessel. In several studies using catheter-based radiation, careful, quantitative coronary angiographic measurements have documented a surprisingly high incidence of inadequate coverage of the injured region by the radioactive source. The balloon catheters used to initially open the stenotic segment can slip forward or backward (“watermelon seeding”), causing unintended injury to the lesion margins. Also, barotrauma from both angioplasty and stent deployment contribute to arterial wall injury beyond the nominal lengths of the balloons or stents (95). Longitudinal seed displacement may also contribute to higher-than-expected restenotic rates at lesion margins owing to the movement of the radioactive seeds relative to the coronary vessel during the cardiac cycle (96). Figure 14.12 is a schematic depiction of the stent and the clinical target in VBT. In addition to more movement in the distal portion of arteries, the movement also varies with the particular artery (eg, possibly more in the circumflex). Uncertainty in target localization can occur because of the difficulty in visual estimation of proximal and distal lesion ends. This uncertainty is compounded by different magnification and obliquity of various projections during fluoroscopy and cine and the relative lack of reference points available (branch vessels are commonly used as reference points for targeting). Finally, the dose falloff and penumbra effect of the particular isotope used can contribute to marginal failure.
Figure 14.11 Schematic cross-sections of the three vascular brachytherapy (VBT) systems. (A) The Checkmate System (J&J, Corp); (B) the Beta-Cath system (Novoste Corp); and (C) the Galileo System (Guidant Corp).
Dose Rate Effects
The biologic consequences of radiation depend not only on the total dose but also on the dose rate at which the radiation is delivered. The absorbed dose measures only the quantity of energy absorbed per unit mass of tissue (Joules per kilogram). The rate at which this dose is delivered has been shown to correlate with the biologic sequelae of the radiation. The dose rate effect in endovascular brachytherapy was investigated using a biophysical model derived from linear-quadratic formalism (86). The goal was to compare the biologic effective doses (BEDs) for different delivery systems. In VBT, the dose rate effect is important for three reasons. First, the radiation is applied close to the source, where the dose rate gradient (falloff) is extremely high, hence the biologic effectiveness varies. Second, different clinical trials utilized different source types, strengths, and designs. In these trials, the same total dose prescription to same radial distance was delivered at different dose rates. The typical dose rate for the WRIST trial (192Ir source) is approximately 50 cG/min at 2 mm radial distance versus 500 cGy/min for the START trial (90Sr/90Y source). Furthermore, different trials prescribe different doses at different radial distances. Third, different source types and designs have different dose gradient profiles; therefore, different dose rates may be delivered.
Figure 14.12 Barotrauma schematic.
1. Spatial relation of target cells and limiting structures. Higher BED in VBT is advantageous if the target tissue is closer to the source than the critical tissues. An ideal situation is demonstrated in the following example in which the target tissue is at 2 mm and the limiting tissue is at 4 mm from the 90Sr/90Y source. The difference in physical dose distribution due to the sharp dose gradient of brachytherapy yields a 4.8 times higher dose to the target (spatial advantage). If the dose rate effect is taken into account, the BED at target tissue is 13.9 times higher (spatial and biologic advantage). This assumption may be substantiated by the preliminary finding that the target cells for restenosis are the endothelial and vessel SMCs located in the coronary vessel, which ranges from 2 to 5 mm in diameter. If this assumption of the relative spatial relation of target and critical structures is correct, the biologic therapeutic window is much greater for VBT than for external beam, and this may explain the fact that the animal data for VBT are much more consistent than that of external beam (97).
2. Dose threshold to cause a late cardiac complication or to inhibit restenosis. The therapeutic window is greater if the dose response curve for restenosis inhibition lies to the left of the dose complication curve. For example, assuming α/β of 3 for cardiac and vascular tissue, if a BED of 5,000 cGy3 is required to cause a complication, and a BED of 1,000 cGy3 is required to inhibit restenosis, then the therapeutic window is great. In contrast, if it takes a BED of 500 cGy3 to cause a complication, then there is an unfavorable therapeutic window.
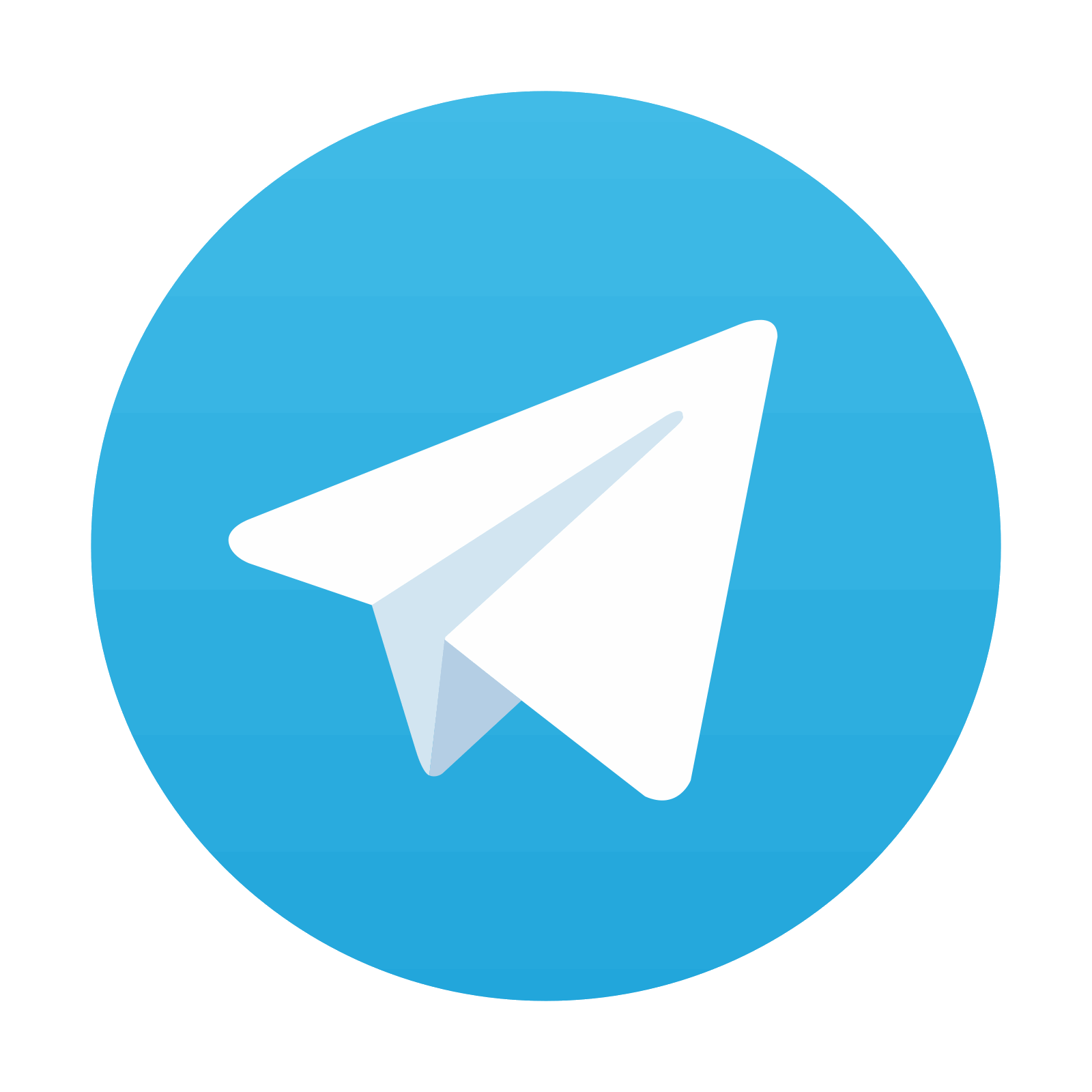
Stay updated, free articles. Join our Telegram channel
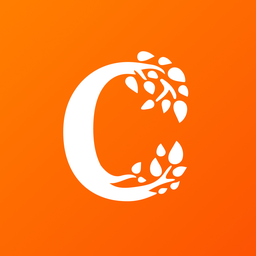
Full access? Get Clinical Tree
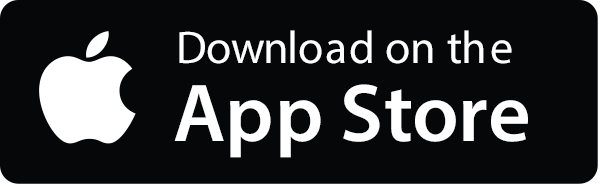
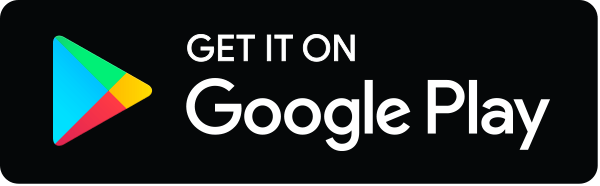