and Ashutosh Dash2
(1)
Nuclear Security and Isotope Division, Oak Ridge National Laboratory, OAK RIDGE, USA
(2)
Isotope Production and Applications Division, Bhabha Atomic Research Centre, Mumbai, India
16.1 Introduction
Targeted radionuclide therapy (TRT) is primarily based on the concept of delivering therapeutic doses of ionizing radiation to disease sites with minimal toxicity to surrounding normal tissues using a target-specific moiety including antibody or antibody fragments, peptides nucleotides, and low molecular weight-specific ligands, linked to an appropriate radionuclide. The expression of intracellular proteins such as nucleolin at the cell surface of tumor cells and tumor endothelial cells emerged as the most common target site because the modality inherently relies on binding to accessible targets on the cell surface rather than overall expression levels. While TRT constitutes a successful paradigm of treating a host of diseases including cancer, the success of this treatment modality is associated with many challenges that include selection and availability of radionuclides with the appropriate half-lives and availability of delivery vehicles capable of incorporating optimal radionuclide activity levels with favorable pharmacokinetics. In addition, suitable tumor biomarkers must be identified which can be used to direct the delivery vehicle to the targeted sites.
Specific tumor-targeting ligands must also be available which can be readily conjugated with the delivery vehicles (Fig. 16.1). Over the years TRT has progressed at a rapid rate and experienced an exponential growth resulting from advances in tumor biology, chemistry, and bioengineered technologies. In order to effectively reach the target site, the radiopharmaceutical must pass through several biological barriers, which include blood vessels, tissues, organs, cells, and even subcellular compartments within the target cell. A targeting strategy based on the specificity of the radiopharmaceutical for the disease site according to its biological function not only precludes the application of every activity levels which are required to achieve sufficient local concentrations, but also must minimize nonspecific toxicity and other adverse side effects.
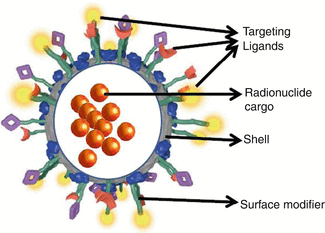
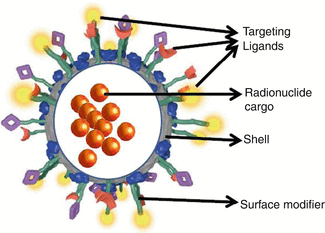
Fig. 16.1
Schematic structural illustration of a nanoparticle comprising the components for radionuclide load delivery
Some of these systems exploit the overexpression of cancer-related surface markers on diseased cells or the development of a dense, but leaky, vascular system within a tumor, forming the basis of a tumor targeting strategy. It is therefore essential to ensure that the radiopharmaceutical exhibits sufficient stability during administration which is maintained for the required time period in vivo for sufficient radiation delivery to the targeted site before catabolism and eventual excretion. A major problem with many of the currently used tumor-targeting vehicles is that a reagent directed to tumor cells will be impeded by insufficient tumor cell to agents in the vasculature. This challenge is particularly prominent with solid tumors, which have a high interstitial pressure, presumably because the blood vessels can be “leaky” and because of poorly functional lymphatic vessels. Radiopharmaceuticals generally do not penetrate further than three to five cell diameters from blood vessels, which can significantly underexpose more distantly located tumor cells to radiation. This phenomenon can then facilitate the development of resistance. Despite rapid advances, the currently available targeting strategies are often limited by insufficient radionuclide delivery to tumor sites due to relatively low and heterogeneous cell surface receptor expression, as well as dose-limiting toxicity to normal tissues. With a view to maximize the therapeutic index and minimize toxicity, it is crucial to deliver radionuclides to the precise site of action with an adequate dose and with appropriate timing. For these reasons, there is a need to develop novel approaches that offer scope for multiple target recognition on cancer cells, at the same time with the ability to intensify the transport of radionuclides with each target recognition event. There is also the potential to deliver radionuclides selectively to more radiosensitive compartments within the diseased tissue.
In this context this chapter discusses in detail the prospects of using nanoparticles (NPs) as vehicles for targeted radionuclide therapy. Such a strategy has generated widespread interest and is particularly appealing since it offers opportunities to overcome the limitations associated with traditional TRT. At the same time, these methods may permit treating disease at molecular level and capability to diminish unwanted side effects of systemic delivery, by increasing tumor accumulation and improving efficacy. Major advantages of using NPs are the ability to create particles functionalized with a wide variety of targeting ligands and physicochemical properties. This versatility permits the formation of agents that are specifically tailored for each application.
16.2 Therapeutic Strategies Based on Nanotargeting
Radionuclide therapy based on the incorporation of radionuclides with specific emission properties into NPs representing a hybrid science emerging from the ramifications of four powerful scientific domains which include material science, bioscience, radiochemistry, and medical applications may represent a new paradigm in an era of image-based radionuclide delivery and dose delivery. These strategies effectively combine use of biological materials, radionuclide therapy, and tools from the basic sciences of physics, chemistry, bioengineering, physiology, and genetics, etc., to fabricate minute synthetic structures. These combined specialties reflect the increasing ability to investigate beyond the molecular level, whereby generating advantageous results that will have significant impact, for instance, for cancer therapy. It is no longer just a promise, but represents the emergence of a new therapeutic modality. Success of nanotechnology in nuclear medicine relies on tumor targeting specificity.
Delivery of radionuclide to a targeted site from nanocarriers can occur by a variety of mechanisms, which include diffusion, particle fusion and cellular internalization, component (lipid–lipid) exchange and convective flux, biolistics, or a combination of these mechanisms. Major advantages of nanosystems to deliver radionuclide pay loads include the ability for preparation in sizes <100 nm and target a specific site, selectively increase the localization of radiopharmaceuticals in the tumor, and deliver the required localized radiation dose while sparing nontargeted tissue, ensuring minimal leakage of radioactive species during circulation. The ability to synthesize NPs functionalized with a wide variety of targeting ligands and physicochemical properties is also important, and this versatility permits the creation of agents that are specifically tailored for specific applications. NPs also have the unique capability to gain access to and even to operate within cells (Wang and Cuschieri 2013; Papa et al. 2012; Astolfo et al. 2013) which offers the opportunity of NPs to interact with subcellular structures. Owing to their small sizes, NPs can extravasate from the intravascular space through the endothelial cell layers and interact with the cell structures of various tissues; however, they are also large enough to transport high payloads of therapeutic or diagnostic agents. Use of NPs offers the capability to use radionuclides of lower specific activity owing to large surface areas.
An additional advantage of nanovectors/nanoparticles is their ability to overcome various biological barriers and to localize into the target tissue. The NP delivery platforms provide a multifunctional capacity that offers the scope for loading multiple moieties, such as targeting ligands and therapeutic agents, using multiple reaction steps. A single NP can also be tagged with various radionuclides for imaging as well as therapy with the concomitant attachment of different targeting ligands to introduce selectivity or active groups for nonradioactive monitoring. The ability to load more molecules or radionuclides on a single particle than conventional carriers is a very unique capability of NPs, since sometimes more than one type of therapeutic agent or radioisotope can be attached. The capability to carry multiple, potentially different, targeting ligands on the nanoparticle can thus provide enhanced receptor binding affinity/specificity. The capability of NPs to bypass biological barriers can enhance targeting efficacy. NP delivery platforms allow for interactions with biomolecules on the cell surfaces and within the cells in ways that unaffected the behavior and biochemical properties of those molecules. NPs generally exhibit prolonged blood retention time, ranging from 30 min to more than 24 h, and the radiolabeled NPs remain stable under many physiological conditions and withstand premature degradation. The flexible chemical properties permit surface modifications to enhance their blood circulation half-life and improve their biodistribution profile, and the use of radiolabeled NPs can offer the capability of preventing premature radionuclide release with subsequent interaction with the biological environment. For these reasons, the use of NPs can improve intracellular penetration and enhance uptake of radionuclides into selected tissue sites, such as solid tumors.
NPs also have the ability to control the pharmacokinetic and radionuclide tissue distribution profile, and internalization of receptor targeted NPs leads to a high payload of radionuclide in the target cells, resulting which is expected to result in effective killing of tumor cells which exhibit only a relatively low level of receptor expression. Unique chemical and physical properties of NPs can also include magnetization and photosensitizing, which provide additional capabilities and functions for improving radionuclide delivery, for example, the external application of a magnetic field and for monitoring the therapeutic response. The incorporation of radionuclides into NPs can be achieved by a variety of methods including chemical surface attachment of a radiotracer via the linkage and/or chelating molecule. The synthesis of NPs uses radiolabeled precursors, and diffusion of radioisotopes into the NPs. They can also be prepared by a variety of other methods, including direct neutron activation, direct ion beam activation, and recoil implantation of radionuclides.
16.3 Selection of Radionuclides for NP Therapy
The selection of a method primarily depends on many factors such as the physicochemical and surface properties of the NPs, NP size, the delivery method to be adopted, the duration of the therapy, among others. While the therapeutic utility of nanocarrier lies at the interface of many disciplines, selection of NP is based on various criteria and depends on many factors such as NP size selection, since nanotechnology techniques allow unprecedented control of the material world, at the nanoscale, providing the means by which materials can be synthesized conforming to desired specifications as well as characteristics. The most desirable size is maintained up to 100 nm to reach tumor affected cells and to provide an opportunity to surpass sinusoid in the spleen and the fenestra of liver Kupffer cells (150–200 nm) and the size of gap junction between endothelial cells of the leaky tumor vasculature (100–600 nm). The chemical composition and structure of NPs are essential to control their interactions. Radiopharmaceutical properties, such as aqueous solubility, stability, etc., can be programmed and loaded. NPs should have an extended circulating half-life, a low rate of aggregation, and a long shelf life. For surface characteristics and functionality, they should exhibit high differential uptake efficiency in the target cells over normal nontargeted cells. For the desired degree of biodegradability and biocompatibility, it is desirable that the NPs are constructed from materials that are biocompatible, well characterized, and easily functionalized. For practicality, the radiation dose delivery profile of the final product should be favorable.
Key properties for radionuclide delivery system using nanocarriers are biocompatibility, proper size and charge to better synergize with the host, high loading and protection of the desired guest molecule, zero premature release before reaching its target, efficient cellular uptake, effective endosomal escape, possible use of ligand on its surface to specifically target tumor cells, stability in circulation, and increasing the fraction of the dose accumulating in the tumor. In order to use a nanocarrier with suitable characteristics for radionuclide therapy, several factors must be considered. These include characterization and extensive evaluation before performing animal experiments since in vivo performance is strongly related to shape, charge, surface modification, and size. The in vivo stability should be scrupulously evaluated using animal experiments since some NPs can be disrupted in the bloodstream. It is also extremely important that the radionuclide chelation with therapeutic agents remains stable over the course of treatment. Therefore, efforts should be focused on preparation of NP-based agents which can allow for efficient, specific in vivo delivery of therapeutic agents without systemic toxicity.
The true power of radioactive therapeutic NPs lies in their ability to interact with disease processes judiciously to deliver the required radiation dose. Radionuclide payload delivery to the target cells from nanocarriers can occur by diffusion, particle fusion and internalization into cells, component (lipid–lipid) exchange and convective flux, biolistics, or some other mechanisms combining these factors. Stability in circulation can be looked up by developing strategies to curtail protein binding and evade the immune system. The efficiency of tumor site accumulation can be augmented by active targeting of the radionuclide delivery system or by increasing extravasation by the enhanced permeation and retention (EPR) effect. The promising attributes of nanomaterials for targeted radionuclide therapy include their ability to concentrate payload of radionuclides for each targeted molecular recognition event in tumors. Two major mechanisms for radionuclide delivery system of NPs to tumor tissue sites are (Allen and Cullis 2004) the site-specific passive tumor targeting and the required molecular affinity and site-specific active tumor targeting for tumor therapy.
16.3.1 Passive NP Targeting
In passive targeting, NPs and radioactive payloads reach the tumor through rich, chaotic, and highly permeable tumor vasculature and are accumulated and subsequently remain in the tumor due to its lack of lymphatic drainage. Accumulation of NPs in tumors takes place due to the pathophysiologic characteristics of tumor blood vessels. When tumor size attains approximately 2 mm3 volume, the increased interstitial pressure within the tumor appears to hinder the diffusion of metabolites and nutrients necessary for tumor growth. As a consequence, a state of cellular hypoxia initiates along with the sprouting of new blood vessels from the established vasculature or angiogenesis to facilitate the supply of oxygen and nutrients to tumor cells to survive and proliferate. The incomplete tumor vasculature results in leaky vessels with enlarged gap junctions of 100 nm to 2 μm, depending on the tumor type, its environment, and its localization. When blood components reach the abnormal, discontinuous vascular bed, the fenestrations offer little resistance to extravasation to the tumor interstitium (Hobbs et al. 1998; Yaun et al. 1995). Owing to the lack a well-defined lymphatic system, such tumors have a compound retention time higher than that of normal tissues (Allen and Cullis 2004; Byrne et al. 2008).
These features provide an enhanced permeability and retention (EPR) effect and emerged as an important mechanism for the passive targeting as well as selective accumulation of NPs in the tumor cell interstitial space. In passive targeting, NPs and payloads could reach the tumor and subsequently remain in the tumor due to its lack of lymphatic drainage. NPs with an optimal particle size offer the opportunity to target the tumor vasculature more effectively where the endothelial barrier has an open fenestration, because normal tissues have tight endothelial junction. One of the ways to enhance EPR is strengthening the stability of nanocarrier to lengthen circulation time, so it will have more opportunities to go through the target position and get together (Torchilin 2002). The tumor accumulation levels depend on factors such as the size of NPs and the leaky vascular pore and the blood circulation half-life, since longer half-lives lead to higher accumulation. In addition, the degree of tumor vascularization, since accumulation is less in poorly vascularized tumors, and the degree of angiogenesis, since poor accumulation in small pre-angiogenic tumors or large necrotic tumors, are crucial.
Among the various requirements for and factors influencing the EPR effect, the most important is having a molecular size larger than 40 kDa (Maeda et al. 1994, 1996, 2003; Doi et al. 1996; Wu et al. 1998, 2001; Tanaka et al. 2003; Matsumura and Maeda 1986). Biocompatibility of the NPs also plays a predominate role. The nanocarrier should not have any interaction with blood components or blood vessels, elicit no antigenicity, retained by the reticuloendothelial (REC) system, and undergo no cell lysis. The luminal surface of blood vessels is well known to bear a negatively charged surface owing to the presence of many sulfated and carboxylate sugar moieties (Maeda 1994). This characteristic dictates that nanocarrier with high positive charges will bind nonspecifically to the luminal surface of vascular walls and be rapidly cleared from the blood circulation (He et al. 2010a, b; Lee et al. 2011). Hydrophobicity of the NPs has an important role. An increase in hydrophobicity of NPs would not only result in higher affinity to a cell membrane (Oda et al. 1986), but also exhibit a much faster endocytotic uptake in parallel with an increase of the cell-association constant to about 10–100-fold (Oda et al. 1986; Oda and Maeda 1987).
However, passive targeting approaches suffer from the limitations that would be expected to obstruct the path toward wide-scale utility. Owing to the large variation on the degree of tumor vascularization and porosity of tumor vessels in different tumor type and status, targeting cancer cells using the EPR effect is not feasible in all tumors (Hobbs et al. 1998; Bae 2009). Internalization of NPs is also hampered by the reduced number of specific interactions of cancer cells. As the passive process of accumulation are not optimally designed toward a biological receptor, a long circulation half-life is required to obtain sufficient drug delivery (Holmberg et al. 2012) and depends largely on the size and surface charge of the NPs (Zhang et al. 2014). Thus, passive targeting is sometimes considered as “nontargeting” (Bae and Park 2011), because the NP carrying radioactive payloads are distributed through leaky vasculature, and is determined by the biological environment, rather than active recognition by the tumors.
16.3.2 Active NP Targeting
In order to circumvent the above limitations, active targeting strategy in which NPs are engineered to attach targeting moieties at their surfaces seemed attractive as the NP scaffold containing target-specific biomarker molecules such as antibodies, proteins, peptides, nucleic acids, sugars, and small molecules such as vitamins provide an opportunity to bind the radionuclide and at the same time offer the scope of targeting the cell surface antigens or membrane receptors that are overexpressed in these cells (Kukowska-Latallo et al. 2005; Weissleder et al. 2005; Liu et al. 2007). While the main mechanism of active targeting lies in the recognition of the ligand by its target substrate, the targeting specificity and the delivering capacity are two important aspects that determine the efficiency of an active targeting system. The targeting specificity is dictated by the ligand as well as NP properties and is decided by the biodistribution of the ligand-functionalized NP and by how the conjugated ligand and the NP system interact with off-target molecules and cells. The delivery of radionuclide payloads to a specific site is directly related to the NP material and structure (Kamaly et al. 2012; Wu and Chu 2013).
Active targeting increases the probability of redirecting long-circulating particles to designated but accessible targets. Unlike passive targeting, in this case, ligands or homing devices that specifically bind to surface epitopes or receptors on the target sites are coupled to the surface of the long-circulating carriers. Strategies for active targeting of tumors primarily involve targeting surface membrane proteins that are upregulated in cancer cells (Huang et al. 2011; Hanahan and Weinberg 2011). Active targeting offers an additional sink for an NP platform since expression of target molecules is usually differential in that the target is highly expressed in tumor cells but expressed at low levels in other cell types in the vascular system. Since the surface area of the vasculature is much larger than the tumor, active binding in tissue can be significant, even for targets that are expressed at relatively low levels. Furthermore, targeting moieties may themselves be targets for receptors on phagocytic cells.
In light of the perceived need to promote and enhance specific interactions between NP and the microenvironment, the surface of particles required to be conjugated with targeting moieties that are recognized specifically by targeted cells. Since NP avidity is dictated by the ligand density, the approaches used to introduce ligands on the surface of the NP represent the cornerstone for the success of actively targeted systems (Gao et al. 2010). The stability of the ligand–NP bond is a key factor that determines how the particle retains the targeting moiety on its surface. While covalent attachment of the ligand is the preferred strategy, physical adsorption using affinity complexes can also be used in case-to-case basis. Owing to the different physicochemical properties of organic and inorganic materials, the type of NP used will determine the difficulty of the ligand conjugation step (Duncan et al. 2005).
16.4 Ligand Conjugation Strategies
Nanoparticles (NPs) can be engineered to target cancer cells for use in therapy. The ultimate goal in the synthesis of multifunctional NPs is the creation of novel NPs for the target-specific therapy. Radiolabeled NPs for radionuclide therapy usually have three major components: core, radionuclide, and targeting biomolecule. The targeting biomolecule serves as a carrier for specific delivery of the radionuclide to the diseased site. With a view to conjugate the ligand to the NP material, both pre-conjugation and post-formulation strategies are being followed. The strategy for the conjugation of biomolecules to nanoparticles generally falls into four classes. Ligand-like binding to the surface of the inorganic particle core is commonly by chemosorptions. Another strategy is electrostatic adsorption of positively charged biomolecules to negatively charged nanoparticles or vice versa. In addition, covalent binding by conjugation chemistry exploits functional groups on both particle and biomolecules, and non-covalent, affinity-based receptor–ligand systems are also exploited.
The choice of the bioconjugation procedure depends strictly on physicochemical and biochemical properties of nanomaterials and ligands. Ligands made by various side chains and residues can interact by multiple coating ligands with the same nanoparticles or even with more nanoparticles. For NP targeting for bioconjugation, one would like to attach the biomolecules to the NPs with control over the following factors, which include the valence or ratio of biomolecule per NP and orientation of biomolecule on the NP, to achieve specific interactions in the final conjugate. Protein, enzyme, and antibody activities are dependent upon their binding sites having access to the environment. The relative separation distance from the NP and attachment affinity must also be assessed, and optimal function and activity of both participants must be maintained. Ideally, the goal should be to replicate in a facile manner with a variety of biologicals.
16.4.1 Pre-conjugation
Pre-conjugation is relatively straightforward one-step formulation procedure that reduces the risks of side reactions and allows greater control over NP properties. Furthermore, this strategy offers the introduction of multiple types of ligands to NPs and facilitates the purification steps subsequent to NP formulation. Pre-conjugation is used when dealing with small molecules, peptides, and aptamers. It is less adapted to native proteins with complex secondary structures as the conjugation step usually involves exposure to organic solvents.
16.4.2 Post-formulation
In this approach the ligands are reacted with formulated NPs. While this strategy works for all types of ligands including antibody, protein, peptide, aptamer, and small molecules, it is preferably used when the stability of the ligand in organic solvents is an issue, the size of the ligand is too large to promote self-assembly, or when the presence of the ligand changes the physicochemical properties of the NP components.
16.4.3 Bioconjugation Based on Covalent Approaches
The formation of covalent NP–ligand conjugates produces stable hybrid systems that provide control over ligand reactivity and NP aggregation. The most popular approach for covalent coupling is based on the existence on proteins of specific and reactive functional groups such as amino, NH2 (lysine); carboxylic acid, COOH (aspartic, glutamic); hydroxyl, OH (serine, tyrosine; and –SH (cysteine) (Weber et al. 2000). Bifunctional linkers are used to conjugate ligands to NPs through a series of chemical coupling reactions. In this case, NPs are functionalized with functional groups such as carboxylic acid, hydroxyl, sulfhydryl, and amino groups. The formation of a peptide bond between the ligand and the NP surface is one approach that is usually achieved by activating carboxylic groups (using, e.g., N-hydroxysuccinimide (NHS) and 1-ethyl-3-(3-dimethyl-amino-propyl) carbodiimide hydrochloride (EDC)) and reacting it with nucleophilic groups (e.g., amine) on the ligand. While this straightforward conjugation strategy can be carried in both aqueous and organic environments, the selectivity of the conjugation and the final orientation of the ligand both depend on the number of nucleophilic functions on the ligand. Carbodiimide coupling is used to covalently link carboxylic acids to amines via formation of a “zero length” amide bond (Gao et al. 2004). The key advantage of this procedure is that it involves no lengthy linker species, allowing the hydrodynamic radius of the NP to be minimized. Charged NPs may be coupled either with oppositely charged biological and polymeric species (Potapova et al. 2003), or indeed to different oppositely charged NPs (Galow et al. 2000).
Another coupling strategy that merits attention is the coupling of a maleimide group on NP surfaces or polymers with a thiol group present on the ligand (i.e., cysteine residues in proteins or peptides) to form a stable thioether bond. In proteins or peptides, reduction of disulfide bonds to free thiol groups allows the reaction. It should be noted that the reduction retain the three-dimensional structure and affinity of the ligand for its substrate intact. While the introduction of free thiols by reacting 2-iminothiolane to primary amines is also a possible pathway which is widely used, this approach introduces a positive charge on the NP–ligand construct (Shi et al. 2009). Recently, a bioconjugation method called “click chemistry” was developed which is a single-step reaction that involves heteroatom bonds with or without catalysts (Singh and Lillard 2009). For example, alkyne groups on peptides or small molecules will readily form a bond with the azide group on the NP surface or polymer backbone without side reactions. This method is very selective and provides very good yields.
16.4.4 Bioconjugation Based on Non-covalent Approaches
Pragmatically, non-covalent conjugation provides a complementary strategy to covalent attachment, enabling applications in sensing and delivery. The simplest way to achieve non-covalent conjugates is to exploit the electrostatic interactions between the nanoparticle and the ligand, a particularly useful approach when specificity is not required (Hong et al. 2004). In order to circumvent the inherent nonspecificity of this approach and to avail regiospecific interaction, the NP surface could be strategically modified by fine-tuning its charge and hydrophobicity (Bayraktar et al. 2007). Interaction between avidins and biotin (bio) is a prominent and widely used non-covalent conjugation technique due to the extremely high affinity of avidins to biotin (dissociation constant 10−15 M (Gao et al. 2010). Choice of biotin is attractive due to its small molecule size which does not alter the functions of the ligands Fischer 2010). Such a strategy appears to be attractive and has been avidly applied to antibodies, peptides, and aptamers (Park et al. 2011) to establish proof of concepts or to screen different ligands without interference from the conjugation chemistry or to study fundamental ligand decoration parameters (Park et al. 2011; Fahmy et al. 2005). While this method constitutes a step forward to achieve non-covalent conjugation, a major limitation of this method is that it may cause immunogenicity due to the presence of the exogenous protein on the surface and it is not amenable for human use (Yumura et al. 2013). In addition, because avidin can bind biotin in a multivalent fashion, cross-linking between the NP constituents is possible. Another popular conjugation strategy is through carbohydrate–lectin interactions (Jiang et al. 2010). Specific carbohydrate–lectin conjugation facilitates uptake via receptor-mediated endocytosis. The preferential uptake of sugar-capped PEGylated QDs by specific asialoglycoprotein has been reported to be successful for in vivo targeting and imaging (Kikkeri et al. 2009).
16.4.5 Influence of the Architecture of Actively Targeted NPs
The conjugation of ligands on the surface of NPs changes not only the properties of targeting molecules but also the nanomaterial (Kamaly et al. 2012; Shi et al. 2011). The ligands lose the rotational and translational freedom bestowed to free molecules, but the new targeted entity achieves improved avidity because of the increased valency. The size, geometry, surface properties (charge and hydrophobicity), and composition of NPs can also be altered. Efficient ligand–receptor interaction for “active targeting” is dependent upon a variety of factors that include (Capone et al. 1984; Li and Hall 2010) the extent of target cell-selective expression of the receptor relative to nontarget cells and the receptor availability on the target cell surface. Furthermore, the rate of internalization versus shedding of that surface receptor following ligand binding is important as well as the expression of a promising tumor-targeting receptor which may not be homogenously distributed within a tumor or may change in its surface expression over time.
16.5 NP Targeting Groups
Targeting ligands with various types of nanomaterials increased specificity and efficacy as well as reduced toxicity. Significantly, the conjugates exhibit special properties, and targeting ligand-conjugated nanomaterials with diverse characteristics display additional capability for therapy (Fig. 16.2). A wide range of ligands may be used as a basis for targeted delivery. Most important parameters that should be considered while selecting a ligand for targeted drug delivery are binding affinity, size, immunogenicity, and cost of production. The ligand should be unique to the target cell and be characterized by the highest binding affinity and lowest immunogenicity. It should also enable penetration of the tumor.
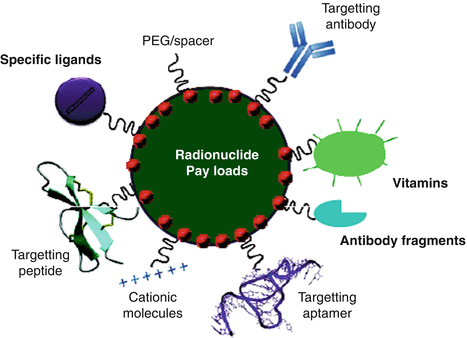
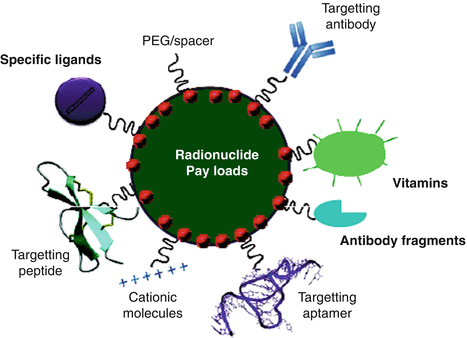
Fig. 16.2
Illustration of the different types of nanoparticle targeting vectors
16.5.1 Monoclonal Antibodies
Monoclonal antibodies (mAbs) possess many desirable technical attributes and advantages as tools for biomolecular targeting, including molecular homogeneity, specificity of interaction, high binding affinity in the nanomolar range, and ease of selection. mAbs is typically used only as a “hook” that facilitates binding of the larger delivery particle with the site of interest on the target cell. The presence of two epitope binding sites in a single molecule offers an exceedingly high selectivity and binding affinity for the target of interest. mAbs represent a single molecular species that bind to antigens with the same affinity and promote the same effector functions. Therapeutic mAbs are usually completely humanized or produced as chimeric proteins, in order to avoid unwanted immune reactions in patients. The binding (dissociation) constants for antibody–antigen interactions vary over a wide range from 10−6 to 10−9 M, but can be as high as 10−12 M for high-affinity antibodies (Dill et al. 1994), Rituximab (Rituxan®) for non-Hodgkin’s lymphoma treatment (James and Dubs 1997), Trastuzumab (Herceptin®) for breast cancer treatment (Albanell and Baselga 1999), Bevacizumab (Avastin®) designed to inhibit angiogenesis (Ferrara 2005), and Cetuximab (Erbitux®) for advanced colorectal cancer treatment (Van Cutsem et al. 2009) have been developed and introduced for clinical therapy.
Antibody-conjugated NPs combine the advantages of NPs with the ability to bind to their target with high affinity and improve cell penetration given by the antibodies. The efficacy of the delivery depends on the ability of each antibody to reach its target in adequate quantities and on the limited amount of nanoparticles trapped by the cell. In order to create a stable linkage, antibodies should be covalently linked to the nanoparticle surface. The physical adsorption method which relies on the affinity of certain functional groups on proteins such as amine and thiol groups and other moieties, for attachment via non-covalent interactions to the particle surface, appeared to be the least stable. In an in vivo environment, or even in cell media, antibodies that are physically adsorbed can be easily displaced. In this premise, the covalent conjugation method which provides a stable linkage between the particle and the antibody and several different chemistries such as N-hydroxysuccinimide (NHS)-coupled NP with 1-ethyl-3-(3-dimethylaminopropyl) carbodiimide (EDC) emerged as the most widely used regime. While the antibody-conjugated NPs is attractive for targeting applications, the F c region of the antibody can be a disadvantage if it is accessible to F c receptors on macrophages, which can lead to increased accumulation in the liver and spleen (Allen 2002) (Fig. 16.3).
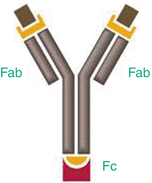
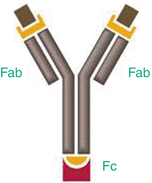
Fig. 16.3
Antibodies are Y-shaped and possess two antigen-binding regions (Fab fragments) and a constant region (F c region)
16.5.2 Antibody Fragments
Although antibodies have proven to be effective targeting agents, there are inherent issues such as decreased receptor affinity due to inadequate conjugation methods, insufficient tumor cell penetration, and nonspecific binding of antibodies to cellular receptors. Being 150 kDa in size, they cleared slowly from the blood pool and lead to significant accumulation in normal organs and relatively limited delivery to lesions such as tumors (Colcher et al. 1998). Antigen-binding sites represent only a small part of the overall size of antibodies. The Fab fragments retain both antigen-binding sites of the antibody coupled by disulfide linkages. Cleavage of the disulfide bond under reducing conditions yields two Fab fragments with sulfhydryl groups that can be used for coupling to the targeting platform. Single chain variable fragments maintain only the variable regions (variable light chain and variable heavy chain) of one arm of an antibody. The use of small fragments of the antibody molecule (Fig. 16.1) such as Fabs (molecular weight MW ~50 kDa; ~6 nm in size) and Fvs (variable region fragments, MW ~25 kDa, ~3 nm in size) offers several advantages over intact antibodies because of the reduced nonspecific binding from F c interactions (many cells have receptors that bind the F c region). The ability to control Fc binding to protein A or protein G is important and allows more efficient penetration of tissue sections. Potentially higher sensitivity in antigen detection in solid phase applications results from the reduced steric hindrance from large protein epitopes. This strategy also allows the elimination of Fc-associated effector functions (e.g., complement fixation) in antigen–antibody binding studies and lower immunogenicity than intact antibody. Finally, these species are cleared much faster from the blood than are intact antibody.
The scope of using Fabs and Fvs fragments is enticing as they can be selected by phage display and are engineered more easily than mAbs, to control properties such as affinity (K D usually lower than 1 nM) or internalization capabilities (Holliger and Hudson 2005). To enable directional attachment, one end of a hetero-bifunctional cross-linker molecule is bound to the F c region of the antibody. The other end of the linker binds directly to the nanoparticle surface, providing a directional linkage between the antibody and the particle. Using this method, a stable linkage between the particle and the antibody is realized while leaving the variable region (F v), or antigen interacting site, sterically unhindered and available for binding. The directional nature of the attachment makes characterization of the antibody-to-particle ratio more consistent and also enhances cell labeling efficiency. Labeling efficiency can be increased by seven to tenfold using a directional versus nondirectional attachment method. It is pertinent to point out that all of these antigen-binding fragments usually retain the specific antigen-binding affinity of the parent antibody, but is usually characterized by improved pharmacokinetics with respect to tissue penetration and lack the Fc-antibody region, which is most immunogenic.
16.5.3 Other Proteins
The three-dimensional shape of proteins offers affinity for specific substrates, and therefore the prospect of using non-antibody proteins (both naturally occurring and synthetic) as targeting moieties seemed possible. The scope of using naturally occurring proteins for therapeutic applications is attractive owing to the presence of endogenous targets. In this premise, transferrin (Tf), an 80-kDa glycoprotein, that regulates the supply of iron into cells via receptor-mediated endocytosis merits attentions (Kresse et al. 1998). On the surface of cells, it binds the internalizing transferrin-receptor (Tf-R) with high affinity The transferrin receptor is expressed at low levels in most normal tissues but is overexpressed in many tumor types (Daniels et al. 2012). NPs decorated with Tf could be used for delivering radioactive payloads. Other proteins, such as human serum albumin (HSA) (Quan et al. 2011), bovine serum albumin (BSA) (Wang et al. 2011), concanavalin A (Smiljanic et al. 2006), protamine (Wang et al. 2012), E-selectin (Rana et al. 2012), and avidin (Li et al. 2013) are just a few promising candidates that could be used for targeted delivery. Synthetic proteins such as “affibodies” (Alexis et al. 2008) or ankyrin repeat proteins decorated with NPs have also been exploited as targeting ligands. This approach seemed attractive as it possesses the advantage of using high affinity, artificial ligands which do not have to compete against highly abundant, naturally occurring proteins.
16.5.4 Peptides
Peptides are formed by joining amino acids together through peptide bonds, which are usually composed of less amino acid than proteins. Peptides offer several unique advantages. Their small size minimizes the overall radius of the resulting peptide–NP conjugate while still affording a high valence (number of peptides per NP) and reduces immunogenicity in vivo. Peptides are also economical and facile to produce as they can be easily synthesized commercially or in the laboratory. From a functional perspective, peptides are biocompatible, derived from naturally occurring protein precursors and can be very specific and bind with high affinity to their cognate receptors, often with affinities comparable to those of full-length antibodies (Yao et al. 2009). Multiple different peptide species can be arrayed around the NP to incorporate multifunctionality or produce a ‘value-added’ material that serves multiple purposes in one NP.
Owing to these attributes, peptides represent a very attractive and useful class of molecules for the development of NPs for targeted delivery. To attach a peptide to an NP and to display peptides uniformly on the NP surface with their active regions all clearly extended away and available for activity, the chemistry involved follows various pathways. These include in a homogenous manner, with control over its final orientation, distance from the NP surface, and the ratio or valence on the NP surface. The strategy used for peptide attachment to an NP will be dictated primarily by a combination of the NP materials themselves, their characteristics, the ligands on their surfaces along with their functional groups, the peptide sequence itself, and the final utility desired.
The most widely investigated peptides in the targeted-delivery applications is probably the integrin-targeting RGD (arginine–glycine–aspartic acid) peptide family (Kamaly et al. 2012; Shi et al. 2011). Integrins αvβ3 and αvβ5 are overexpressed on tumor endothelium and some epithelial cells during tumor growth, angiogenesis, invasion, and metastasis and constitute an interesting molecular target for a tumor-homing approach. The binding affinities of some of the RGD-containing derivatives for αvβ3 range from 3.2 to 100 nM (Huang et al. 2008; Alloatti et al. 2012). The addition of specific amino acid residues to peptide sequence motifs, such as RGD, that induce binding to cell-attachment proteins, strongly enhances the binding affinity of this peptide. In this premise, RGD peptide functionalized Au–Fe2O3 developed by Tang’s group for cancer cell-specific apoptosis and real-time imaging merit attention (Gao et al. 2012). Among other targeting moieties, cytokines and typical peptides released from a variety of cells during an inflammatory response which exhibit broad biological activities should also be mentioned. They are produced by immune cells (such as monocytes, macrophages, T cells, B cells, NK cells, etc.) and some nonimmune cells (endothelial cells, epithelial cells, fibroblasts) after stimulation. Shenoi and coworkers found tumor necrosis factor α (TNF-α), a representative cytokine, has the potential to improve tumor-specific action in combination with locally applied thermal therapy in prostate cancer when conjugated to AuNPs (Shenoi et al. 2013). The agonist or antagonist pharmacologic activity of peptides has been harnessed to alter the fate of NPs decorated with such peptides (Hild et al. 2010). In this study, the targeting of quantum dots to G-protein-coupled receptors using peptide agonists led to the internalization of the nanomaterial.
16.5.5 Aptamers
Aptamers are single-stranded deoxyribonucleic acid (DNA) or ribonucleic acid (RNA) oligonucleotides that can bind to target antigens with high affinity and specificity. Target molecules for aptamers can be virtually any class of substrate ranging from whole cells to large molecules, like proteins, to peptides to drugs and organic small molecules or even metal ions. Aptamers have a molecular weight (10–15 kD) which is one order of magnitude lower than that of antibodies (150 kD) (White et al. 2000) and derive their name from the Latin word “aptus” meaning “to fit.” Owing to their small size and similarity to endogenous molecules, aptamers exhibit superior tissue penetration (Hicke and Stephens 2000) and are believed to be less immunogenic than antibodies (Drolet et al. 2000). In comparison with antibodies, these molecules have some advantages because of their molecular nature which offers the scope for storage at room temperature owing to their high stability (especially DNA aptamers). They can be heated to temperatures as high as 95 °C or exposed to various solvents or harsh environments and will return to their original confirmation, providing a long shelf life (White et al. 2000) and low immunogenicity and toxicity. Chemical modifications are also possible to extend their lifetime in biological fluids, to immobilize them on surfaces, to endow them with radionuclide (Jenison et al. 1994; Lee et al. 2006), and to “tune” their half-lives to match the indication (Carlson 2007). The low molecular weights facilitate penetration of these molecules into tissues and cells.
While the attributes of aptamers are appealing, these molecules are susceptible to nuclease degradation or renal clearance in vivo. Therefore, their pharmacokinetic properties need to be enhanced prior to in vivo applications. Although aptamers themselves are typically quite good at homing to their intended targets even in complex solutions including the in vivo milieu, nanoparticles can be used for directing aptamer NPs and radioactive payloads to their target cells. In view of their small sizes, they require attachment to the nanoparticles via linkers sufficiently long to ensure that they are not sterically hindered from contacting their specific targets. Conjugations to nanoparticles have been extensively studied and reviewed (Teply et al. 2006; Farokhzad et al. 2006; Ellington et al. 2007). Aptamer-conjugated NPs target cancer cells of different types, including prostate cancer (Lupold et al. 2002; Farokhzad et al. 2004; Wang et al. 2007; Zhang et al. 2007) and breast cancer (Huang et al. 2009). With specificity for integrins, they can target tumor vasculature (Schiffelers et al. 2004).
16.5.6 Vitamins
Vitamins such as vitamin B9 (folic acid) and vitamin H (or vitamin B7, biotin) offer the scope for targeting diseased tissue. In this premise, folic acid (FA), a low-molecular-weight vitamin which plays an essential role in cell survival and binds with high affinity to the folate receptor (FR), a membrane-anchored protein that is overexpressed on the surface of a variety of human tumors including ovarian, brain, breast, colon, renal, and lung cancers merits attention (Low et al. 2008: Hilgenbrink and Low 2005; Markert et al. 2008). The prospect of using folate-conjugated NP is enticing because of low-cost, nonimmunogenic character and ability to conjugate with a wide variety of NP and ability to enter cells through receptor-mediated endocytosis (Pan et al. 2013). Folate-targeted NPs co-encapsulating paclitaxel and yittrium-90 showed improved survival in a xenograft model of ovarian cancer. Riboflavin is an essential vitamin primarily responsible for cellular metabolism, and the riboflavin carrier protein (RCP) is highly upregulated in metabolically active cells (Foraker et al. 2003). With these data in mind, flavin mononucleotide (FMN), an endogenous RCP ligand, could be used as a targeting ligand for metabolically active cancer or endothelial cells.
16.5.7 Specific Ligands
Biotin is a CO2 carrier for several important biological reactions, including gluconeogenesis and the synthesis of fatty acids, and the metabolism of amino acids acts as an aid in maintaining blood sugar levels in people and promotes the health of sweat glands, nerve tissue, bone marrow, male sex glands, blood cells, skin, and hair. Conspicuously, biotin levels might be correlated with diseases such as diabetes mellitus, liver and skin disorders, immunological and neurological abnormalities, and epilepsy (Ho and Hung 2008). Biotin has been successfully incorporated into the architecture of carbon nanotube (CNTs) to develop a multifaceted delivery vehicle with high stability, substantial cell viability and targeted specificity toward cancer cells (Brahmachari et al. 2014). Among the synthetic and natural small molecule ligands reported, triphenylphosphonium (TPP) and its derivatives have been used for mitochondria targeting (Marrache and Dhar 2012; Smith et al. 2003). The TPP molecule is a cationic, relatively large, and hydrophobic molecule that can penetrate easily through the cell membrane. Despite the desire to direct therapeutics to the mitochondria, the actual task is more difficult due to the highly complex nature of the mitochondria. The potential benefits of integrating nanomaterials can lead to the development of nanomedical platforms for targeting therapeutics to the mitochondria. An investigation indicated that the positively charged TPP could accumulate several hundredfolds within mitochondria (Marrache and Dhar 2012).
Carbohydrates (Zhang et al. 2010a, b, c) are a large class of small molecule targeting ligands which can selectively recognize cell surface proteins receptors, such as lectin (André et al. 2000). The asialoglycoprotein receptor (ASGP-R) is present on hepatocytes at a high density of 500,000 receptors per cell (Shen et al. 2011; Kim et al. 2005). It has a tendency to bind carbohydrates including galactose, mannose, and arabinose and therefore can thereby serve as an effective liver-targeting ligand (Yang et al. 2010; Cho et al. 2008) Hyaluronic acid (HA), a copolymer of N-acetyl D-glucosamine and D-glucuronic acid, can be effectively used for targeting macromolecule that can bind to cluster determinant 44 (CD44), which is overexpressed in various tumors (Gunthert et al. 1991; Platt and Szoka 2008; Bhang et al. 2009).
16.6 Radiolabeling
The ultimate goal in the synthesis of multifunctional NPs is the creation of novel NPs for the target-specific therapy. The radiolabeled nanoparticles for tumor targeting usually have three major components: core, radionuclide, and targeting biomolecule. The targeting biomolecule serves as a carrier for specific delivery of the radionuclide. The radionuclide can be conjugated directly on the surface of nanoparticle core through various labeling methods. In order to obtain optimal therapeutic outcome, appropriate therapeutic radionuclide and radiolabeling strategy must be carefully taken into consideration. Some key factors need to be considered while undertaking radiolabeling of nanoparticle. In designing a radiolabeled nanoparticle several key factors must be considered including radiolabeling integrity. The nanoparticle structure and radiolabeling strategy must both be designed to get robust, stable radiolabeling. The half-life of the radionuclide must be congruent with the binding kinetics of the carrier and target, as well as the in vivo pharmacokinetics of the carrier. The targeting efficiency and radiolabeling-specific activity, for well-designed nanoparticle allows increased loading of targeting ligands and high radiolabeling-specific activity and can provide elevated binding efficiency to reduce the required administration of nanoparticle to just trace amounts. The radionuclide can be conjugated directly on the surface of nanoparticle core through t various labeling approaches, including (Xing et al. 2014) direct labeling (nucleophilic or electrophilic reactions), indirect labeling (through prosthetic group), coordination chemistry labeling of chelator-functionalized NPs, and nucleophilic halogen exchange chemistry. Among these approaches, complexation reactions of radiometal ions with chelates through coordination chemistry have been widely used (Fig. 16.4).
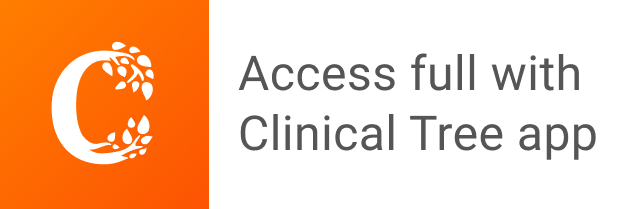