Introduction
Post-surgical evaluation of bone and joints is critical to guiding clinical care following trauma and joint replacement, as it allows for assessment of fracture healing, infection and prosthesis failure or complication. While radiographs are the mainstay technique for postoperative imaging, CT and MRI provide critical problem-solving tools for the assessment of recurrent injury or complication.
Magnetic resonance imaging (MRI) and computed tomography (CT) of bones and joints following orthopedic intervention present both technical and diagnostic challenges due to imaging artifacts related to implanted hardware and metallic shavings, as well as post-surgical scarring and anatomic alteration. While the presence of orthopedic hardware may reduce the image quality and anatomic visibility, it should not be regarded as a contraindication to performing CT or MRI. Additional artifacts may arise due to additional technical and physical factors resulting in degraded image quality and, in some situations, simulated pathology. Musculoskeletal radiologists should be able to recognize the appearances of imaging artifacts, particularly those related to orthopedic hardware, and be familiar with CT and MRI artifact reduction techniques.
Computed Tomography
Conventional polychromatic computed tomography (CT) employs a fan-shaped beam of radiation traveling through the patient as he or she is moved through the imaging gantry on an automated table. Images may be obtained at various slice thicknesses. A projection of this beam as it passing through the subject is recorded using a detector array, and multiple projections are acquired from the same table position to create a single axial image. Resulting images are reconstructed using a filtered back projection algorithm, and both spatial and contrast resolution are affected by the selected filter algorithm, such as bone or soft tissue.
The axial CT images may be reformatted in various planes with most musculoskeletal CT studies including axial, sagittal, and coronal reformations oriented along the anatomic axes of the imaged structure with additional reformations of varying obliquities to evaluate specific anatomic features, such as the glenoid fossa, acetabulum or femoral head–neck junction. The density of the imaged structures affects the CT appearance, with more-dense structures resulting in greater beam attenuation. They will consequently appear “brighter” or white, while less-dense structures, such as fat or gas, will appear “darker” or black.
The presence of very high-density material, such as metal, will markedly attenuate the x-ray beam, prohibiting some radiation from reaching the detector altogether. The highly attenuating metal results in beam-hardening, excessive quantum noise, photon starvation, scattered radiation, and edge effects with consequent beam alteration (Li et al., 2009). The loss and alteration of x-rays due to metal results in distortion of the projection data and will appear on reconstructed CT images as artifact, such as streaking and shadowing (Buckwalter et al., 2011; Watzke & Kalender, 2004) (Figure 8.1). Further, multidetector CT scanners have resulted in new image artifacts as a result of incomplete sampling in the z-axis (table motion) direction, which is termed splay or windmill artifact, and appears as rotating lines surrounding regions with high-contrast boundaries, particularly about bone and soft tissue or lung (Flohr et al., 2005). Given the high attenuation of metal, splay artifact is substantially exaggerated at the margins of implanted hardware.
Figure 8.1 Streak and shadow artifact. (A) Axial CT image of the lumbar spine demonstrates extensive high-attenuation linear streak and splay artifact secondary to posterior spinal fusion hardware. (B) Axial CT image of the left hip demonstrates linear low-attention shadow artifact propagating along the axis of greatest metal cross-section, which obscures the femoral neck.
Conventional Postoperative CT Imaging
Given the effects of metallic hardware on the quality of CT imaging, concerted effort is required to optimize studies by minimizing artifact. Various factors affect the severity of metallic artifact, including hardware features, such as alloy type and geometry, and technical factors including kVp, mAs, pitch setting and image reconstruction algorithm.
A variety of metal alloys are employed for surgical hardware, and the degree of beam attenuation varies depending on the hardware composition. While it is not possible to ascertain the type of specific alloy used for the implanted hardware, the expected degree of attenuation can be assessed on the initial scout image. Highly attenuating (or dense) hardware will appear bright white on initial scout images or prior radiographs, while less-attenuating metallic hardware will appear gray (Figure 8.2). The most radiopaque and attenuating hardware consists of stainless steel and cobalt chrome alloy, which was commonly used in older total hip prostheses (Buckwalter, 2007). Titanium appears much less radiopaque and is currently used in new femoral prostheses. Zirconium implants will appear less radiopaque than cobalt, but more radiopaque than titanium (Buckwalter et al., 2011). If the area of interest for the CT study does not include the implanted metallic hardware, the field of view and imaging plane can be adjusted. In addition, if the hardware is in the contralateral extremity, such as with knee or ankle implants, the imaged extremity can be extended while the contralateral extremity is flexed reducing the effect of the hardware on the quality of the CT images.
Figure 8.2 Variable hardware attenuation. (A) CT scout image demonstrates heterogeneous density of glenoid components of reverse total shoulder arthroplasty. (B) Axial CT image shows metallic glenosphere (curved arrow) positioned appropriately on less-dense metaglene (straight arrow).
The size and geometric configuration of the metallic implant substantially impacts the appearance of the associated streak artifact. Implants with round transverse cross-section, such as intramedullary nails, will result in a uniform projection independent of beam orientation with minimal artifact on reconstructed images. On the other hand, implanted hardware with asymmetric cross-sectional geometry, such as a thin but wide metallic plate, will result in propagation of streak artifact along the longest cross-sectional axis. The presence of perpendicular transfixing screws will further increase artifact.
Complex cross-sectional geometry implants will result in streak artifact along the axes of greatest cross-sectional thickness (Buckwalter et al., 2011). In the presence of multiple implants the artifact will be most severe along the axis containing both implants. For example, with bilateral hip arthroplasties, the artifact will be most pronounced along the right–left axis as the beam travels through both implants (Figure 8.3). By orienting the hardware with shortest dimension of the implant perpendicular to the table, the amount of associated streak artifact will be reduced.
Figure 8.3 Artifact summation. (A) Scout image demonstrates bilateral total hip arthroplasties. (B) Axial CT image at the level of acetabular cup and femoral head shows severe transverse streaking related to total summed attenuation of metal components.
Basic CT technical factors can be adjusted to reduce the effects of beam attenuation due to metallic hardware with greater benefits in the presence of smaller hardware, such as suture anchors or scaphoid screws (Buckwalter et al., 2011; White & Buckwalter, 2002). First, beam energy may be increased to 140 kVp, as opposed to standard 120 kVp. This modification may increase the patient radiation dose by 20%–30%, and caution should be exercised when imaging radiation-sensitive regions, such as the pelvis, neck, and chest; however, distal extremity imaging may be performed without concern for significant increase in total body dose (Biswas et al., 2009; Nickoloff & Alderson, 2001). Following this when employing multislice CT scanners, mAs can be increased when scanning through regions containing hardware while utilizing a low pitch setting. This combination of increased mAs and kVp will further increase radiation exposure. Close attention should be paid to dose modulation techniques, and technical adjustments should be made to minimize radiation exposure while obtaining diagnostic-quality images.
Splay artifact may also be addressed using a lower pitch setting on multislice CT imaging. By increasing the thickness of reconstructed slices, the appearance of splay artifact decreases and will be essentially eliminated when the slice thickness is two or more times greater than the detector element thickness (Buckwalter et al., 2011). Therefore, it is typically desirable to reconstruct axial slices thicker than the minimum, particularly in routine pelvic imaging.
It is important to consider that thinner slices will reduce the effect of metallic artifact, and a reduced pitch will be useful to handle splay artifact in this setting. In addition, z-axis focal spot dithering (or flying z spot) technique can also address splay artifacts by acquiring additional samples along the z-axis to fill in undersampled data without requiring decreased pitch or resulting in compromised imaging quality (Flohr et al., 2005).
Bone (or “detail”) filters use a very sharp image filter, which accentuate fine spatial details, including image noise and artifact. Because musculoskeletal CT images are typically reviewed using “bone” windows (window 2000 HU, level 500 HU) these fine artifacts may be obscured and overlooked (Figure 8.4). In the presence of metallic hardware, these artifacts occur to a much greater extent and can result in degraded image quality. In the setting of metallic implants, it is helpful to use a soft image reconstruction, such as the standard filter used for general abdominal imaging, and even softer filters can be considered in the setting of highly attenuating implants, such as cobalt or steel, or if there is concern for soft tissue abnormality adjacent to hardware (Buckwalter et al., 2011). Evaluation of fine hardware in a small region of interest, such as a scaphoid fracture, offers an exception to this rule where a sharp bone algorithm may be beneficial (Ohashi & El-Khoury, 2009).
Figure 8.4 Optimal windowing. (A) Axial CT image of the pelvis viewed in soft tissue windows demonstrates extensive streak artifact due to total hip arthroplasty prohibiting evaluation of adjacent bone and soft tissue structure. (B) Same axial CT image set to bone windows results in improved visibility of adjacent bone and soft tissue anatomy.
When performing multiplanar reformations, it is important to consider that metallic streak artifact will degrade the quality of reformatted images, particularly when created using minimum pixel thickness, which is the default setting. By increasing the reformat thickness, the severity of hardware artifacts is reduced, and a slice thickness between 1 and 2 mm will provide adequate artifact reduction without exaggerated partial volume averaging (Buckwalter et al., 2006, 2011).
Three-dimensional volume rendering techniques may also be useful to reduce hardware-associated streak artifact (Calhoun et al., 1999; Fayad et al., 2009). However, even so, the presence of severe streak artifact may result in rendering of unacceptable streaking limiting the quality and utility of the three-dimensional reconstruction. Given this, three-dimensional volume rendering may be most useful for evaluation of metal hardware rather than adjacent bone (Figure 8.5).
Figure 8.5 Periprosthetic 3D reconstruction. (A) 3D reconstruction of osseous anatomy surrounding right total shoulder arthroplasty without dual-energy technique demonstrates significant artifact limiting evaluation of hardware and glenohumeral joint. (B) Dual-energy CT 3D reconstruction focused on metallic hardware demonstrates excellent visualization of hardware with metaglene screw fracture (arrow).
Dual-energy CT and Metal Artifact Reduction
Recent advances in CT imaging have permitted the application of dual-energy CT (DECT) theories, which were first considered in the 1970s (Chiro et al., 1979). Simultaneously acquiring two CT data sets using two beams at separate kilovolt peaks, most commonly 80 and 140 kVp, from the same anatomic location, allows computation of differences in attenuation values within a structure and determination of the dual energy index (DEI) (Coupal et al., 2014). This dual-energy processing obtains material-specific information and has been applied in many imaging applications, including diagnosis of gout (Nicolaou et al., 2010), automatic bone removal (Johnson et al., 2007), virtual unenhanced imaging (Takahashi et al., 2008), and renal stone composition characterization (Boll et al., 2009). At present, this technology is limited to specific DECT scanners and cannot be performed using conventional CT technology, which continues to be the greatest limitation of this technique. Table 8.1 shows a sample DECT protocol.
In addition to these useful applications, virtual monochromatic images may be synthesized using the data from the dual-energy scans with the benefit of reduced beam-hardening artifact (Alvarez & Macovski, 1976). Moreover, the high photon energies in DECT reduce the effects of photon starvation with improvement in streak artifact (Coupal et al., 2014). These virtual monochromatic images appear as if created at a polychromatic, or wide, spectrum of energy levels. Using post-processing tools, the virtual monochromatic energy level of the image can be varied allowing a windowing effect. This permits the radiologist or technologist to find the ideal setting where the energy is high enough to overcome metal artifact, but not obscure adjacent soft tissues, and is typically in the range of 105–140 keV (Coupal et al., 2014; Lee et al., 2012; Meinel et al., 2012;). As with conventional methods, the metal artifact is reduced, but not completely eliminated (Figure 8.6).
Figure 8.6 Dual-energy CT. (A) Axial CT image acquired with dual-energy technique demonstrates minimal artifact secondary to sacroiliac joint screws. (B) Coronal multiplanar reformation demonstrates excellent visualization of the osseous anatomy and hardware with further minimization of artifact.
Numerous studies have reported that DECT is an effective method of reducing metal artifact and improving diagnostic quality when directly compared to conventional polychromatic CT. An additional benefit of DECT is the ability to obtain images with improved diagnostic quality and reduced artifact without an increase in total radiation dose (Bamberg et al., 2011; Johnson et al., 2007; Pessis et al., 2013). This stands in comparison to the previously discussed methods of increasing kVp and mAs for conventional CT imaging.
Along with limited availability and cost of DECT technology, there are additional shortcomings that will be continued to be addressed as the technique is further developed. First, virtual monochromatic reconstruction requires omission of lower energy photon data to extrapolate high energy levels, resulting in noisier images (Coupal et al., 2014). Further, processing of DECT data can only be performed using a soft-tissue algorithm with associated loss of high spatial frequency detail found in bone. At present, dual-energy images cannot be reconstructed from the CT scanner’s raw data set in coronal and sagittal planes. Consequently, multiplanar reformations can only be produced from image data on the workstation or with post-processing software, resulting in further loss of tissue detail. Finally, despite the advantages of DECT, large or extensive dense metallic implants continue to generate significant metallic artifact resulting in degraded imaging quality (Figure 8.7) (Yu et al., 2012).
Figure 8.7 Suboptimal dual-energy CT. (A) Frontal radiograph demonstrates comminuted, intra-articular distal humeral fracture following open reduction and internal fixation. (B) Dual-energy CT ordered to assess fracture union provides suboptimal visualization of the intra-articular fracture site secondary to extensive, persistent artifact resulting from summation of multiple fixating screws.
Postoperative CT Imaging Applications
With the continued refinement of CT metal artifact reduction techniques, the modality has seen increased usage for hardware evaluation, particularly for associated complications, and progression of fracture healing. The interpreting radiologist should be familiar with the common indications for postoperative CT imaging to add further diagnostic value to image interpretation and ensure performance of the optimal examination to address the clinical question.
Immediate and Subacute Postoperative Complications
Radiographs remain the modality of choice to evaluate immediate postoperative appearances of fracture fixation and joint arthroplasty and confirm adequate fracture reduction and appropriate hardware placement. CT is uncommonly employed for acute postoperative extremity evaluation, but it may be considered following reduction of an intra-articular fracture or hardware placement, such as an external fixator, when planning another intervention or considering revision (Ohashi et al., 2009).
In contrast, due to the complexity of spine anatomy, pedicle screw placement in the spine is more effectively imaged with CT, which is 10 times more sensitive in the detection of medial pedicle cortex violation (Farber et al., 1995). Accurate screw placement with CT can help predict postoperative outcomes, as pedicle screw canal perforation less than 4 mm on images reformatted perpendicular to the screw is associated with no neurologic complication (Laine et al., 1997). In addition to spinal cord or nerve root compromise, additional paraspinal injuries may be diagnosed more readily, including pneumothorax and hematoma.
Chronic Postoperative Complications – CT Evaluation
Periprosthetic Fracture
While periprosthetic fractures may occur acutely or chronically following fracture fixation or arthroplasty, CT may be more beneficial in the chronic setting to accurately assess the exact extension of the fracture and residual bone volume, which may be obscured by overlying surgical hardware (Figure 8.8) (Ohashi et al., 2009). Further, CT may be helpful to better assess multiple overlapping screws or complex hardware before surgical intervention. Cross-sectional imaging with CT or MRI may also be useful for the assessment of incomplete or stress fractures resulting in chronic postoperative pain.
Figure 8.8 Periprosthetic fracture. (A) Coronal multiplanar reformation and (B) axial CT image of the right hip demonstrate radiographically occult non-displaced periprosthetic fracture involving the greater trochanter (arrow).
Non-union
Fracture healing and joint fusion may be assessed readily with radiographs, but the presence of callus formation alone does not predict progression to complete healing, and the presence of osseous bridging is considered a more reliable indicator of union (Figure 8.9) (Ohashi et al., 2009). CT has been found to be more accurate for the determination of osseous bridging, because radiographs may either underestimate or overestimate the extent of bone fusion (Grigoryan et al., 2003; Krestan et al., 2006). The presence of exuberant callus formation may obscure the evaluation for osseous bridging on radiographs, and multiplanar CT allows direct visualization of the fracture site. In addition, fine central osseous bridging at the site of small fractures and bone grafts, such as in treated, non-united scaphoid fractures, is better assessed with thin-slice CT images (Figure 8.10). Finally, some articulations are difficult to assess due to overlapping anatomy, such as the posterior subtalar and Lisfranc joints, and CT offers superior delineation of the joint spaces following arthrodesis.
Figure 8.9 Fracture union. (A) Sagittal multiplanar reformation demonstrates non-united distal fibular fracture. (B) Follow-up dual-energy CT coronal multiplanar reformation demonstrates distal fibular fracture healing after fixation for non-union.
Figure 8.10 Scaphoid fracture evaluation. (A) Sagittal multiplanar reformation shows central portion of compression screw in the scaphoid with incomplete union at periphery of fracture site (arrowheads). Note absence of streak artifact due to small size of screw. (B) Coronal multiplanar reformation shows fracture union at the central portion of the scaphoid waist, indicating partial union.
Osteolysis
Periprosthetic osteolysis represents a host of conditions, including small-particle disease, mechanical hardware loosening and periprosthetic infection, manifested by bone destruction surrounding implanted hardware (Figure 8.11). Imaging may not be able to differentiate the underlying cause of osteolysis, but identifying its extent and potential associated soft tissue involvement provides useful information to the referring physician.
Figure 8.11 Hardware infection. Coronal multiplanar reformation of the shoulder demonstrates post-surgical changes following removal of infected left total shoulder arthroplasty with placement of antibiotic spacer and glenoid osteolysis (arrowheads) due to ongoing infection.
In the case of infection, which is uncommon, nuclear medicine studies, aspiration or biopsy may be required to confirm the diagnosis. Small-particle disease results from implant wear, leading to particle shedding and provocation of a histiocytic response, which manifests as bone destruction. The most common cause of small-particle disease is polyethylene wear, and most patients remain asymptomatic until extensive bone loss is present (Naudie & Rorabeck, 2004; Ohashi et al., 2009). Radiographic evaluation of small-particle disease can be limited by location of the lesion, position of the hardware, and patient body habitus. In particular, evaluation of peri-acetabular particle disease can be challenging due to the above factors. In such cases, CT may be indicated to determine the presence and volume of osteolysis prior to hardware revision (Figure 8.12) (Chiang et al., 2003; Claus et al., 2004).
Figure 8.12 Small particle disease. Axial CT image of the pelvis demonstrates left total hip arthroplasty with adjacent cortical disruption (curved arrow) and destructive soft tissue lesion (arrowheads) arising from the anteromedial aspect of the acetabulum, consistent with small particle disease due to polyethylene wear.
Evaluation of osteolysis requires attention to optimized metal artifact reduction with use of a soft tissue kernel and wide window settings (> 6000) to best visualize periprosthetic lucency (Ohashi et al., 2009). Focal lucency greater than 2 mm or interval increase compared to prior CT studies typically indicates osteolysis and should prompt clinical evaluation for an underlying cause (Ohashi et al., 2005; Taljanovic et al., 2003). It is important to note that comparison of periprosthetic lucency measurements between radiographs and CT may differ and small differences should not be relied upon for diagnosing osteolysis. CT may also be used for prosthesis templating prior to revision arthroplasty in cases of severe bone loss and remodeling.
Hardware Failure
Radiographs remain the gold standard for assessing the integrity of implanted hardware; however, CT may be useful in particular cases. Prior to complete fracture union, hardware failure poses a significant impediment to fracture healing, and, in fact, may be an indicator of fracture non-union as chronic mechanical stress at the persistent fracture site results in hardware fracture. Consequently, CT examinations for fracture healing should also include a close inspection of surgical hardware, particularly in cases of non-union.
Just as with the immediate postoperative setting, CT is the modality of choice for evaluating complications related to spinal fusion. Screw fracture may occur in up to 25% of patients (Figure 8.13) (Lonstein et al., 1999; Ohashi et al., 2009). As with all cases of hardware failure, the imaging findings should be correlated with patient symptoms as not all hardware complications relate to current clinical symptoms. CT can be useful for the detection of polyethylene dislocation, which may occur in shoulder, hip and knee arthroplasties, and may be difficult to detect on radiographs (Figure 8.14) (Clarke et al., 2004).
Figure 8.13 Hardware failure. Sagittal multiplanar reformation demonstrates fractured pedicle screw in patient with treated vertebral compression fracture and sudden onset of worsening back pain.
Figure 8.14 Polyethylene liner displacement. Sagittal multiplanar reformation of reverse total shoulder arthroplasty demonstrates posteriorly displacement of low attenuation polyethylene liner relative to the humeral component and glenosphere in patient with limited range of motion.
Magnetic Resonance Imaging
Postoperative MR Imaging
As opposed to CT, MRI employs radio waves to create images, and the main magnetic field within the MR scanner establishes a setting which facilitates the absorption and emission of radiofrequency energy from the various tissues throughout the body. Generation of MR images relies on interactions between the magnet, radiofrequency (RF) transmitter and receiver, and gradient coils, as well as an image reconstruction algorithm, to accurately encode spatial localization of MR signal.
Optimal imaging requires a homogeneous magnetic field, and the complex interplay of these components and the imaged patient can result in a multitude of imaging artifacts, particularly in the presence of metallic hardware (Buckwalter et al., 2011; Zhuo & Gullapalli, 2006). By recognizing the appearance of these MR artifacts, potential corrective actions can be undertaken to improve image quality and diagnostic value.
Motion Artifact
MR image reconstruction techniques ideally require a completely stationary patient, and motion artifacts are the most common artifact in imaging of the musculoskeletal system as random patient motion and the motion secondary to various physiologic processes, such as breathing, swallowing and peristalsis, is seldom completely controlled during examinations (Singh et al., 2014).
Motion artifact occurs secondary to the inability of the phase gradient to predictably encode radio waves arising from moving structures (Peh & Chan, 2001). The type of motion, speed of movement, and magnetic field strength determine the severity of motion artifact, and higher field strength magnets result in more substantial artifacts (Taber et al., 1998). Image ghosting and smearing are the common artifacts arising from both voluntary and involuntary patient motion (Figure 8.15) (Wood & Henkelman, 1985). The most effective method to reduce motion-related artifact is imaging with pulse sequences that shorten acquisition time, reducing the time interval in which patient motion may affect image quality (Singh et al., 2014). This has been accomplished with technical advances in MRI by use of stronger gradients, multichannel coils, and rapid image acquisition and reconstruction techniques, such as single-shot and parallel imaging (Morelli et al., 2011).
Figure 8.15 Motion artifact. Degraded image quality of sagittal T1-weighted fat-saturated post-contrast MR image of the foot due to patient motion.
Ensuring patient comfort with an appropriately sized coil equipped with soft pads between the inner surface of the gradient coil and the patient’s skin while securing the limb with immobilizing devices, such as Velcro straps, will also reduce motion artifact (Singh et al., 2014). If motion persists despite comfort measures, imaging may be suspended for patient reassurance and conscious sedation may be considered in select patient populations, such as claustrophobic subjects.
Repetitive, periodic motion can also occur due to cardiac activity or vascular pulsation and result in ghost images propagating along the phase-encoding direction (Peh & Chan, 2001). Pulsatile motion amplitude and speed will determine the brightness of this artifact (Wood & Henkelman, 1985). Cardiac or respiratory gating, as well as use of short acquisition times, may be used to minimize the effects of this periodic motion artifact; however, these artifacts are more problematic in cardiac and abdominal MR imaging than in musculoskeletal MR imaging (Singh et al., 2014). Pulsation artifact from the popliteal artery is a common manifestation of this artifact in musculoskeletal imaging, and may result in obscuration of the menisci (Figure 8.16).
Figure 8.16 Pulsation artifact. Axial T2-weighted fat-saturated MR image of the pelvis demonstrates femoral artery pulsation resulting in ghost images (arrowheads) obscuring the anterior labra and simulating femoral head lesions bilaterally.
Similarly, CSF pulsation is another source of motion artifact resulting in phase-encoding directional ghosting within the spinal canal, which may obscure evaluation of the spinal cord and even simulate intradural lesions or mimic vascular flow voids (Rubin et al., 1988; Singh et al., 2014;). In certain cases, the absence of CSF pulsation artifact has been reported to provide a useful indicator of cord compression (Quint et al., 1989). This artifact responds poorly to standard periodic motion correction techniques, and is best addressed with use of gradient-echo sequences, which are typically avoided in post-hardware imaging (Larsen et al., 1996; Peh & Chan, 2001).
Wraparound Artifact
This common MR artifact, also known as aliasing, occurs when imaging with a too small field of view (FOV) that is insufficient to cover the tissues being imaged. The resulting artifact appears as a superimposition of the phase-encoded signal from outside and within the FOV giving resultant wraparound of structures on the opposite side of the image (Figure 8.17). Wraparound artifacts are always encountered in the phase-encoding direction as the MR system oversamples in the frequency encoding direction (Zhuo & Gullapalli, 2006).
Figure 8.17 Wraparound artifact. Axial proton-density-weighted MR image of the right knee demonstrates wraparound artifact (straight arrow) of the left knee, which is partially imaged (curved arrow).
Wraparound artifact may be simply addressed by employing a larger FOV, but this will result in a reduction of image quality. Saturation pulses can be used to exclude undesirable signal arising from structures outside the FOV, and oversampling techniques can be employed without reduction in image quality (Peh & Chan, 2001). With oversampling, the number of phase-encoding steps is automatically doubled along with the FOV along the phase-encoding direction, and the resultant increase in scan time is compensated for by halving the number of excitations with a preserved signal to noise ratio (Arena et al., 1995). In addition, switching the frequency and phase-encoding direction and imaging with a rectangular FOV can also reduce wraparound artifact. Finally, some MR units are equipped with a “no phase wrap” technique, which can eliminate aliasing by doubling the FOV in the phase-encoding direction and automatically cropping the image to a final square FOV (Singh et al., 2014; Taber et al., 1998).
Truncation Artifact
Truncation artifact is typically seen at tissue interfaces with an abrupt change in intensity of the MR signal and is also referred to as Gibbs phenomenon or ringing (Czervionke et al., 1988). This artifact occurs due to undersampling of the several phase-encoding steps of high spatial resolution and is manifested by dark or bright lines occurring parallel to the periphery of the region with abrupt signal intensity shift (Singh et al., 2014). Truncation artifact commonly occurs at fat–muscle and CSF–spinal cord interfaces and has been reported to simulate spinal cord syrinx or atrophy, intervertebral disc abnormalities, and meniscal tears (Figure 8.18) (Breger et al., 1988; Bronskill et al., 1988; Levy et al., 1988; Singh et al., 2014; Turner et al., 1991). Imaging with at least 192 phase-encodings steps, as well as a smaller FOV with increased matrix size along the phase-encoding direction, can reduce truncation artifacts (Peh & Chan, 2001). As with wraparound artifact, truncation may be reduced by switching the phase- and frequency-encoding directions.
Figure 8.18 Truncation artifact. (A) Sagittal STIR MR image demonstrates T2 hyperintense line (arrowheads) within the anterior spinal cord due to spinal-cord CSF interface. (B) Corresponding axial MEDIC (multi-echo data image combination) MR image demonstrates normal appearance of the spinal cord.
Shading Artifact
Shading artifact is also known as intensity gradient artifact. It occurs due to non-uniformity of the RF field, resulting in loss of voxel signal intensity in regions of low RF strength, and is frequently seen in the setting of imaging with a surface coil (Hyde et al., 1987; Singh et al., 2014). This artifact results in variable image contrast, loss of brightness, and subsequent deterioration of image quality due to decreased RF signal strength in structures located further from the coil, and can be corrected by use of an enclosing coil or a larger surface coil (Figure 8.19). The presence of this artifact should prompt a change in coil used for the examination, such as a dedicated extremity coil, if possible.
Figure 8.19 Shading artifact. (A) Incomplete coil coverage results in dark signal obscuring the soft tissues adjacent to the olecranon at the periphery of sagittal STIR image of the forearm in a patient with cellulitis and concern for abscess. (B) Axial T1-weighted post-contrast fat-saturated image obtained following coil repositioning demonstrates abscess (arrow) in the previously obscured region.
Radiofrequency Interference/Zipper Artifact
This MR imaging artifact results from leakage of electromagnetic waves into the MR imaging suite, which can be detected by the receiver coil and interfere with imaging processing or recording (Zhuo & Gullapalli, 2006). The consequent image distortion appears as a region of increased noise with linear bands of variable signal intensity traversing the image perpendicular to the frequency-encoding direction.
Sources of RF interference can include electronic devices, fluorescent lights, static discharge, radio broadcasting stations and imaging hardware malfunction (Figure 8.20) (Peh & Chan, 2001). If identified during imaging, the technologist should ensure that the door to the MR suite is tightly sealed to prevent entry of external RF waves, and if the artifact persists, particularly on multiple examinations, the integrity of the suite’s RF shield should be investigated (Singh et al., 2014).
Figure 8.20 Radio-frequency interference artifact. Zipper-like linear bands of increased signal intensity are seen on axial T1-weighted MR image of the hand.
Susceptibility Artifact
Susceptibility artifact is the most important artifact occurring in postoperative musculoskeletal imaging, and the occurrence of this artifact is highly dependent on the type of implant or device being imaged. Magnetic susceptibility represents a measure of the extent of magnetization of an object placed in an external magnetic field, and is variable for different tissues and directly proportional to magnetic field strength. Susceptibility artifact results from local magnetic field inhomogeneity at the interface of structures with differing magnetic susceptibilities.
The severity of field inhomogeneity depends upon the interfacing structures, and is most severe adjacent to ferromagnetic materials, such as joint prostheses, fracture fixation hardware, metallic implants, dental prostheses, surgical clips, and metallic foreign bodies. The presence of metal implants result in large variations in the precession rate (or resonant frequency) across the object, giving signal loss due to dephasing, resulting in blooming artifact, as well as displacement artifacts. The associated blooming artifact due to signal loss manifests as a black area where there would otherwise be signal distortion of anatomy (Figure 8.21A). Displacement artifacts occur in the slice selection and readout directions and include geometric distortion, signal loss, and signal pile-up, compounding the artifact due to dephasing.
Figure 8.21 Susceptibility artifact. (A) Sagittal proton-density-weighted fat-saturated image of the knee shows susceptibility artifact arising from three metallic screws for patellar tendon transfer with obscuration of the patellar tendon insertion and proximal tibial marrow. (B) Sagittal proton-density-weighted image of the knee shows blooming dark signal (arrow) secondary to intra-articular gas following traumatic arthrotomy.
Along with image distortion and dephasing artifact, the metallic susceptibility artifact results in a shift in resonance frequency of lipid and water resulting in failure of fat suppression, which can obscure soft tissue and marrow edema (Figure 8.22). Certain fat-supression techniques are more sensitive than others. The most common method employed for suppression is to employ chemically selective saturation. This technique, typically referred to as fat saturation, relies on the fact that fat resonance is 220 Hz below that of water at 1.5 T to selectively suppress fat. In the setting of metallic hardware, surrounding frequency shifts can range from 3 to 80 kHz, resulting in substantial changes in the adjacent fat resonance leading to loss of fat suppression as the saturation pulse completely misses the new resonant fat frequency.
Figure 8.22 Failed fat suppression. Coronal T2 fat-saturated image of the ankle with extensive susceptibility artifact due to distal fibular plate and screws shows failed marrow fat suppression involving the distal tibia and hindfoot with demarcation between successful (arrow) and failed suppression in the talus (curved arrow).
While large metallic implants may substantially degrade image quality as described above, smaller metallic foci can mimic pathology as a result of blooming artifact. Fine metallic debris in postoperative patients can mimic spinal stenosis or bone proliferation as bone and metal both can appear hypointense on certain sequences. In addition, soft tissue and intra-articular gas can result in susceptibility artifact, and it may be difficult to distinguish fine metallic debris from gas in the setting of recent surgery or infection (Figure 8.21B). Gradient echo sequences can also result in susceptibility artifact where blooming manifests as apparent enlargement of bone with adjacent soft tissues appearing diminutive. The presence of susceptibility artifact can significantly obscure pathology in the postoperative patient and diagnostic evaluation requires dedicated measures to reduce the artifact.
Metal Artifact Reduction Techniques
Despite the severity of artifact due to metallic hardware, there are many techniques that can be combined with careful parameter selection to acquire diagnostic images in the postoperative setting. First, as with CT, it is important to understand that the degree of image distortion is dependent on the atomic and molecular structure of materials composing the hardware, as well as the net magnetic field associated with their atoms.
The majority of materials can be classified as diamagnetic, paramagnetic, superparamagnetic, or ferromagnetic (Mitchell, 1999). Iron, nickel, and cobalt are examples of ferromagnetic metals used in orthopedic and spine implants, which result in significant magnetic field distortion and most severe susceptibility artifact (White & Buckwalter, 2002). To reduce the effects of susceptibility artifact, more implants are being manufactured using paramagnetic metal alloys, like zirconium and titanium, which do not retain magnetization in the absence of an externally applied field and result in significantly less susceptibility artifact (Beltran et al., 2014). In fact, it has been shown that there is more confidence, less variability, and greater interobserver agreement in the evaluation of periprosthetic structures in the setting of zirconium knee arthroplasty compared to cobalt–chrome hardware because of decreased artifact (Raphael et al., 2006).
Metallic orthopedic hardware will induce artifact occurring both in the plane that is being imaged (in-plane artifact) and in adjacent planes (through-plane artifact) due to magnetic susceptibility and eddy currents within the hardware (Lu et al., 2009). When imaging in the presence of orthopedic hardware, there are several MRI parameters which can be modified to reduce susceptibility artifact. First, scanning should be performed at a lower magnetic field strength, as metal-induced artifact is proportional to magnetic field strength with a 3 T magnet producing twice as much susceptibility artifact as a 1.5 T magnet; however, imaging should not be avoided if only a 3 T magnet is available.
Overall, the following parameter alterations should be made: increased bandwidth, enlarged matrix size, reduced slice thickness, and lower time-to-echo. By employing a high readout bandwidth, geometric and in-plane distortion, including signal pile-up and loss, are reduced. In addition, the phase-encoding directions should be swapped to reorient the area affected by artifact, which will also serve to reduce in-plane distortion (Potter et al., 2004; Sofka et al., 2003).
Distortion effect may also be reduced by increased slice-selection bandwidth. Increased slice-selection bandwidth will result in increased power deposition (or specific absorption rate), which may necessitate longer repitition times (TRs) or fewer interleaved slices per repetition (Hargreaves et al., 2011). An increased readout bandwidth will result in reduced signal-to-noise ratio (SNR), but this parameter alteration offers a very effective and easy metal artifact reduction technique.
The amount of spatial distortion in the through-slice direction is the ratio of frequency offset to slice bandwidth multiplied by the slice width. By using thinner slices, the amount of through-plane distortion will be reduced, but this is at the cost of increased scan time to cover the region of interest, as well as reduced SNR secondary to decreased voxel size. If possible, the region of interest can be altered to image a smaller region to maintain a shorter scan time. Multiple slices can be averaged during post-processing to reduce effect on SNR.
To reduce signal loss from dephasing, which occurs due to rapid variation in the static magnetic field, spin-echo techniques, including FSE, TSE, and RARE, should be employed. These sequences use a 180° refocusing pulse that reverses static field dephasing. Alternatively, ultrashort echo time (UTE) methods may be considered as they avoid the signal loss in tissues with short T2 relaxation time between excitation and readout seen with spin-echo imaging. They instead employ incredibly short echo times, which minimize time to readout allowing less time for magnetization to diphase, improving structural visualization (Beltran et al., 2014).
The use of spin-echo methods is the simplest method to dramatically reduce metal-induced artifacts (Hargreaves et al., 2011). Spin-echo sequences can be combined with different radiofrequency bandwidths for excitation and refocusing pulses resulting in varied displacement for excited and refocused slices in the presence of metal-induced frequency offsets. While spin-echo methods can suppress displacement artifacts, they cost further SNR decrease, as well as potentially increased scan times and larger slice gaps (den Harder et al., 2015; Hargreaves et al., 2011).
As discussed, many metal artifact reduction techniques require a SNR tradeoff, and specific MR imaging techniques have been designed to reduce metal-induced susceptibility artifact while maintaining a high SNR and providing diagnostic fluid-sensitive fat-suppressed images. One such sequence is referred to as multiple-acquisition with variable resonances image combination (MAVRIC), which uses multiple spectrally overlapping nonspatially selective 3D acquisitions to reduce encoding errors present in individual Fourier reconstruction (Choi et al., 2015; Koch et al., 2009). Unfortunately, the non-spatially selective excitation results in long acquisition times (den Harder et al., 2015).
Another metal artifact reduction sequence is referred to as slice encoding for metal artifact correction (SEMAC), which employs view angle tilting (VAT) to ameliorate readout encoding distortions and adds additional phase-encoding steps in the slice-dimension to re-register slice distortions, resulting in correction of both in- and through-plane distortion (Lu et al., 2009).
The WARP sequence is a combination of SEMAC, VAT, and increased bandwidth and can be performed as either a T1-weighted or STIR (short tau inversion) sequence (Sutter et al., 2012). As opposed to the purely frequency-selective MAVRIC, SEMAC is spatially selective with spatial shifts due to frequency (Beltran et al., 2014). A combination MAVRIC–SEMAC sequence (MAVRIC-SL) has been developed and tested in animal models with superior lesion detection and artifact reduction compared to conventional FSE sequences (Liebl et al., 2015). Further, combined UTE–MAVRIC sequences are being investigated with the aim of improving the detection of postoperative complications related to short T2 tissues adjacent to metal implants, such as osteolysis and tendon/ligament injuries (Carl et al., 2013).
Despite water excitation working well in the presence of metal, effective fat suppression poses a challenge in the postoperative patient due to the alteration of resonant frequency of adjacent fat. The most common fat-suppression technique, chemically (or spectrally) selective fat saturation, should be avoided. In particular, gradient-recalled echo (GRE) and spin-echo spectral fat saturation should be avoided, as they will exaggerate susceptibility artifact not only adjacent to the implanted hardware, but also obscure a large portion of the FOV.
The preferred method for fat suppression during metal artifact reduction imaging is STIR imaging, which relies on the short T1 relaxation time of fat to null its signal. This technique is much less sensitive to magnetic field variation and provides homogenous fat suppression near metal, but is limited by low SNR ratio due to attenuation of non-fat signal by the inversion pulse, as well as incompatibility with contrast-enhanced imaging, which relies on short T1 relaxation of contrast agents (Figure 8.23).
Figure 8.23 Inversion recovery imaging. (A) Axial spectral adiabatic inversion recovery (SPAIR) image used during MR examination of the abdomen and pelvis demonstrates large regions of susceptibility due to bilateral metal-on-metal hip arthroplasties as this technique is highly sensitive to field inhomogeneities. (B) Axial STIR MR image in the same patient reveals fluid-signal pseudotumor (arrow) adjacent to the left greater trochanter, which was obscured on prior examination.
Dixon techniques may also be considered, but are thought to be less effective than STIR imaging, as these techniques can track gradual magnetic field variations and provide reasonable fat saturation at a distance from the implanted hardware; however, Dixon techniques will typically fail to suppress fat signal in tissues adjacent to the metal. Given the limitation of STIR imaging, Dixon-based methods likely represent the best option for fat suppression during contrast-enhanced imaging with an understanding that suppression surrounding the hardware will likely fail. See Table 8.2 for a sample metal artifact reduction MR imaging protocol.
Table 8.2 Sample metal artifact reduction MR imaging protocol.
Sequences | |||
T1 FSE | Axial | Sagittal | Coronal |
STIR | Axial | Sagittal | Coronal |
Positioning | Long axis of hardware along magnetic field | ||
Scanning | Frequency gradient direct away from region of interest, employ FSE and wide receiver bandwidth (16 or 32) | ||
Matrix | 512 × 512 | ||
Do not use | Intravenous contrast, gradient echo sequences, standard fat-saturation | ||
If available | Use proprietary metal artifact reduction techniques (MAVRIC, SEMAC) |
Applications of MRI
As with CT, MRI can be performed to assist in the evaluation of infection, mechanical loosening, hardware failure, or periprosthetic fractures. While CT is the preferred cross-sectional imaging technique for investigating osseous anatomy, MRI provides more detailed assessment of soft-tissue structures. Familiarity with common postoperative indications for MRI allows the interpreting radiologist to tailor the examination to adequately assess the structures of interest and to refine the search for postoperative complications.
Adverse Reaction to Metal Debris
Metal-on-metal hip arthroplasties were initially introduced in the 1930s, but fell out of favor with the introduction of metal-on-polyethylene hardware (Ostlere, 2011; Wiles, 1958). The unsatisfactory long-term results of metal-on-polyethylene implants in younger patients, specifically related to liner wear and associated small-particle disease, have led to a resurgence of metal-on-metal prostheses with improved design (Ostlere, 2011; Sochart & Porter, 1997). However, hip arthroplasties with metal-on-metal components are subject to unique postoperative complications owing to implant composition and biologic response (Hayter et al., 2012). Metallosis results from shedding of large quantities of metallic debris. It is more common in metal-on-metal hip arthroplasties, but may also occur following failure of polyethylene liners. This condition results in cloud-like regions of opacity on radiographs, and hip aspiration will reveal oily, black fluid (Bestic & Berquist, 2013).
Another adverse reaction to metal debris is described histologically as aseptic lymphocyte-dominated vascular-associated lesions (ALVAL), a delayed hypersensitivity response to metal particles. Affected patients will present as early as a few months following surgery with a painful palpable mass, which may be accompanied by femoral neuropathy (Clayton et al., 2008). This condition is associated with regions of adjacent tissue necrosis of varying size believed secondary to ingestion of microscopic cytotoxic metal particles by macrophages (Mahendra et al., 2009; Ostlere, 2011). These reactive lesions can appear predominantly solid with regions of necrosis, mimicking the appearance of a malignant neoplasm, thus leading to the descriptive term “pseudotumor” (Pandit et al., 2008).
The formation of periprosthetic pseudotumors is now recognized as a major cause of metal-on-metal hip arthroplasty revision and raises long-term safety concerns about such implants (Ostlere, 2011). The risk factors for developing a pseudotumor include female sex, small prosthetic cup size, poor component positioning and sizing with increased frequency in large femoral head implants, as well as 33% chance of contralateral pseudotumor in bilateral resurfacing arthroplasties (De Haan et al., 2008; Glyn-Jones et al., 2009; Grammatopoulos et al., 2010; Ostlere, 2011). Elevated serum and intra-articular metal ion levels (cobalt and chromium) are associated with this condition, and it is important to correlate the imaging findings with the patient’s laboratory parameters (Kwon et al., 2011).
On MRI, pseudotumors will appear as solid, cystic or mixed masses adjacent to and possibly communicating with the hip joint with potential intralesional or peripheral low T2 signal, reflecting metal deposition (Figure 8.24) (Chen et al., 2011). Solid lesions more commonly occur anteriorly, usually within the psoas muscle, and may extend proximally into the pelvis (Figure 8.25) (Ostlere, 2011). Predominantly cystic lesions typically arise from the posterior joint space and may or may not demonstrate wall-thickening. Lateral lesions usually involve the trochanteric bursa and often communicate with the joint space. Differentiation of cystic and solid lesions may be difficult and the use of post-contrast imaging is not helpful for lesion detection, particularly due to surrounding metallic artifact. There may be involvement of the adjacent osseous structures with associated erosions, as well as the soft tissues, particularly the gluteal tendon attachments with resulting tendon disruption and muscle atrophy (Figure 8.26) (Anderson et al., 2011). In rare cases, the reactive process may involve pelvic or lower extremity arteries or the femoral nerve, particularly when occurring anteriorly (Chen et al., 2011; Clayton et al., 2008). At present, there is no established pathway for the management of metal-on-metal pseudotumor patients, and follow-up imaging may be performed to assess lesion progression before proceeding to revision, especially with small lesions or minor symptoms.
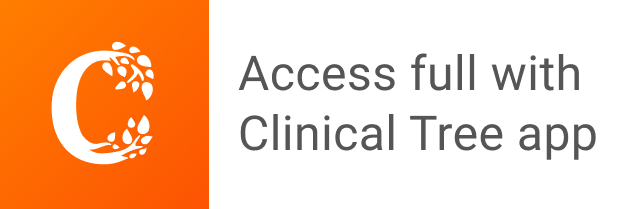