Advances in MR and computed tomography (CT) techniques have resulted in greater fidelity in the assessment of treatment response and residual tumor on one hand and the assessment of recurrent head and neck malignancies on the other hand. The advances in MR techniques primarily are related to diffusion and perfusion imaging which rely on the intrinsic architecture of the tissues and organ systems. The techniques exploit the density of the cellular architecture; and the vascularity of benign and malignant lesions which in turn affect the changes in the passage of contrast through the vascular bed. Dual-energy CT and CT perfusion are the major advances in CT techniques that have found significant applications in the assessment of treatment response and tumor recurrence.
Key points
- •
Posttreatment morphologic alterations cause a significant change in the morphology and function of head and neck structures.
- •
Advanced magnetic resonance imaging (MRI) and computed tomography (CT) techniques have increased the accuracy in the detection of residual and recurrent lesions.
- •
Advanced techniques need to be tailored for the locoregional assessment of the head and neck.
- •
A summary review of these techniques is presented with highlights of the recent studies on the advances in imaging techniques in evaluating posttreatment sequela.
Introduction
Anatomic localization and preoperative functional assessment are the 2 important foundations for successful outcomes in the treatment of head and neck cancers. Given the complexity of the soft tissues including the vascular, neural, and lymphoid structures, the aim of the successful management of these cancers involves the trans-spatial excision of one or more groups of these structures followed by a combination of radiotherapy, chemotherapy, or both. Excision of the soft tissue structures often entails reconstruction using transposed, interposed material, or free flaps. Each of the other above-mentioned therapeutic interventions causes profound changes in the anatomy of the region. These morphologic alterations can obscure tumor recurrence. Advanced computed tomography (CT) and magnetic resonance imaging (MRI) techniques have, therefore, been developed to assess for posttreatment sequela and to assess for residual and recurrent disease ( Tables 1 and 2 ). Advances in both CT and MRI techniques form the basis of the initial assessment of the patients undergoing treatment for head and neck malignancies.
Scan Type | Helical Noncontrast CT |
---|---|
Gantry Rotation Time/Length | 0.8s Full |
Detector Coverage | 40 mm |
Slice Thickness | 2.5 mm |
Interval | 2.5 mm |
Speed | 20.62 |
Pitch | 0.516:1 |
KVP | 120 |
mA | 80 |
DVOV | 18 |
Scan Type | Cine – CT Perfusion |
---|---|
HiRes Mode | Off |
Gantry Rot Time/Length | 1.0s Full |
Detector Coverage | 40 mm |
Slice Thickness | 5 mm |
# of Images/Rotation | 8i |
Cine Time b/w Images | 1.0s |
Scan Duration | 50s |
Interval | 0 |
KVP/mA | 120/65 |
DFOV | 25 |
Delay | 5s |
Vendor Neutral Parameter | Sample 3T Value |
---|---|
Coil | At least 16 channel |
Parallel Imaging | On |
Sequence | 3D T1 weighted |
TR/TE | 4.91/1.91 |
Flip Angle | 12 degrees |
Slice Thickness | 4.0 mm |
Slices Per Slab | 36 |
Field of View | 230 mm |
Temporal Resolution | 8.85s |
Number of Sections/Scan | 40 |
Total Scan Time | 6:07 min |
Anatomic considerations
Squamous cell carcinomas are the most common malignancies encountered in the head and neck. Management of these cancers in the nasopharynx, oropharynx, hypopharynx, and larynx is often multimodality-based. Lesions in the sinonasal region and the oral cavities are primarily surgically excised and treated by adjuvant radiation or chemotherapy. Additionally, surgical treatment involves the removal of draining lymph nodes. Nodal metastases occur along the expected regional drainage pathways and a thorough knowledge of these locations is helpful in managing these patients. Nodal anatomy and the lymphatic pathways are nearly always distorted following surgery and radiotherapy, and this may result in challenges in locating metastases that may not correspond to the lymphatic drainage territories of the resected tumor.
CT and MRI remain the mainstay in the primary diagnosis of head and neck cancers. Multidetector CT and MRI with conventional sequences have been used for the initial preoperative staging of both the primary tumor and the nodal anatomy. Modification to the conventional techniques for the regional assessment of malignancies has been described.
Surgical interventions can be broadly classified into neck dissection for lymph nodes, reconstructions of the postsurgical defects using, or a combination of both procedures. Surgical procedures can be followed by radiation treatment or radiation treatment may be the sole method of treatment, as in the case of early laryngeal carcinomas.
There are many types of neck dissection including radical, modified radical, selective, and extended radical neck dissections that involve either the removal or sparing of multiple normal adjacent structures such as the ipsilateral sternocleidomastoid muscle, spinal accessory nerve, and internal jugular vein. A detailed description of these types is beyond the scope of this article and has been dealt with in another article in this issue.
Radiation therapy induces significant morphologic and histopathological alterations of the cutaneous, subcutaneous, muscular, and glandular structures of the neck, which ranges from early edematous changes and later fibrotic sequelae giving the neck the characteristic woody feel on palpation. Changes following radiation therapy are most pronounced during the first few months after the end of radiation treatment and are visualized on both CT and MRI. They include the reticulation of the subcutaneous fat and deep fat planes, with the thickened appearance of the skin and platysma muscle. Enhancement and thickening of the pharyngeal walls and edema in the retropharyngeal space together with increased enhancement of the major salivary glands can also be observed. Later sequela includes atrophy of the salivary glands (postirradiation sialadenitis) and atrophy of the lymphatic tissues. Surgical reconstruction of the postsurgical defects involves the placement of flaps composed of skin, subcutaneous fat, fascia, with or without muscle; and usually a vascular pedicle. Detailed descriptions of the complications of chemoradiation and the imaging appearances of different types of flaps and their contents are presented in a different article in this issue.
Pathology and advanced imaging techniques
Magnetic Resonance Diffusion
MR diffusion sequences have been designed to measure the Brownian motion of water molecules in tissues exposed to strong uniform magnetic fields. The random motion of water molecules in tissues is impeded when they encounter cell membranes, cellular organelles, and nerve fibers. Similarly, increased cellularity and cytotoxic edema associated with tumors hinder the motion of water molecules. Quantification of diffusion is performed by inbuilt software algorithms and the resulting value is termed as apparent diffusion coefficient (ADC), which is expressed in millimeters squared per second. Values for solid tumors in the head and neck vary depending on the cell type. Malignant lesions have a lower value than benign tumors and lymphomas have a lower value than carcinomas. ADCs values for lymphomas are reported to be between 0.5 and 0.9 × 10 −3 mm 2 /s, for thyroid cancers they are higher at 1.3 to 3 x10 −3 mm 2 /s, and for squamous cell carcinomas they have an intermediate value of 0.6 to 1.5 × 10 −3 mm 2 /s with a mean of 0.9 to 1.2 × 10 −3 mm 2 /s. Poorly differentiated squamous cell carcinomas have lower values than well-differentiated carcinomas. Lower ADC values have been attributed to hypercellularity, hyperchromatism, and a higher nuclear-to-cytoplasm ratio. The limitation of routine use of ADC values lies with the images being often motion degraded by breathing and swallowing, and due to single-shot EPI sequences which are frequently used for the acquisition of these sequences. A workaround for greater fidelity of the calculation of ADC values is to use multiexponential models with several B-values, with higher b values of 800–1000 s/mm 2 being more suitable for the accurate assessment of these parameters.
Detection of larger metastatic lymphadenopathy is more easily accomplished by conventional CT or MRI; however, diffusion imaging can help in determining metastatic lymphadenopathy in subcentimeter nodes (less than 10 mm in diameter). This may have important implications while evaluating the postirradiated neck whereby small metastatic nodes may be impalpable. For the detection of impalpable metastatic nodes, a study has revealed an ADC threshold of 1.0 × 10 −3 mm 2 /s leading to a high sensitivity of 92.3% and a specificity of 83.9% as a discriminator of benign and metastatic nodes.
Differentiation of recurrent and residual tumors from posttreatment sequelae can be made with reasonable accuracy by diffusion imaging. Morphologic distortions following surgery and radiation therapy with and without chemotherapy may result in extensive fibrosis and scarring on follow-up. Biopsies in these treated areas for the suspicion of tumor recurrence often result in equivocal results. Repeat surgical intervention may induce further radiation necrosis. Continued surveillance is, therefore, key in the assessment of recurrence which most frequently occurs at the site of the primary lesion. Diffusion-weighted imaging was noted to help in the differentiation of chronic postradiation sequelae and recurrence based on the ADC measurements in this situation. Changes in the ADC values were seen early and late in the posttreatment course with a high sensitivity of 94.6% and specificity of 95.9%. Recurrent lesions characteristically appear as low-intensity areas on ADC maps than posttreatment scarring which tends to show higher signal intensities. The predictive value of ADC maps in the postoperative assessment for head and neck squamous cell carcinoma recurrence was studied by Brenet and colleagues. The authors compared ADC values in patients up to 8 days before the commencement of chemotherapy and 3 months after the completion of chemotherapy. In a univariate analysis, the initial ADC values were significantly lower in patients presenting with residual tumors (0.56 ± 0.11 vs 0.79 ± 0.13; P <.001). In disease-free individuals followed-up for additional 12 months after chemotherapy, the only factor which was significantly correlated with disease recurrence was the relative change in the ADC values. Studies by Kim and colleagues, however, showed that ADC values initially were lower (1.04 ± 0.19 × 10 −3 mm 2 /s) in patients who responded favorably to chemoradiation than in partial responders (1.35 ± 0.30 × 10 −3 mm 2 /s). They also found a significant increase in the ADC values in tumors which showed complete response as early as 1 week after the commencement of the treatment. In another study by Vandecaveye and colleagues, the authors compared the ADC values before and after the treatment, and concluded that the change in ADC values before and after treatment.
(Δ ADC) for recurrent tumors was significantly lower than those who showed complete remission for the primary lesion (−2.3% ± 0.3% than 80% ± 41%; P = .0001). These studies underline the importance of DWI in both the diagnosis, treatment, and follow-up of head and neck cancers. Moreover, the importance of this technique is further enhanced with assessment with conventional MRI sequences, as was shown by Tshering Vogel and colleagues ( Fig. 1 ).
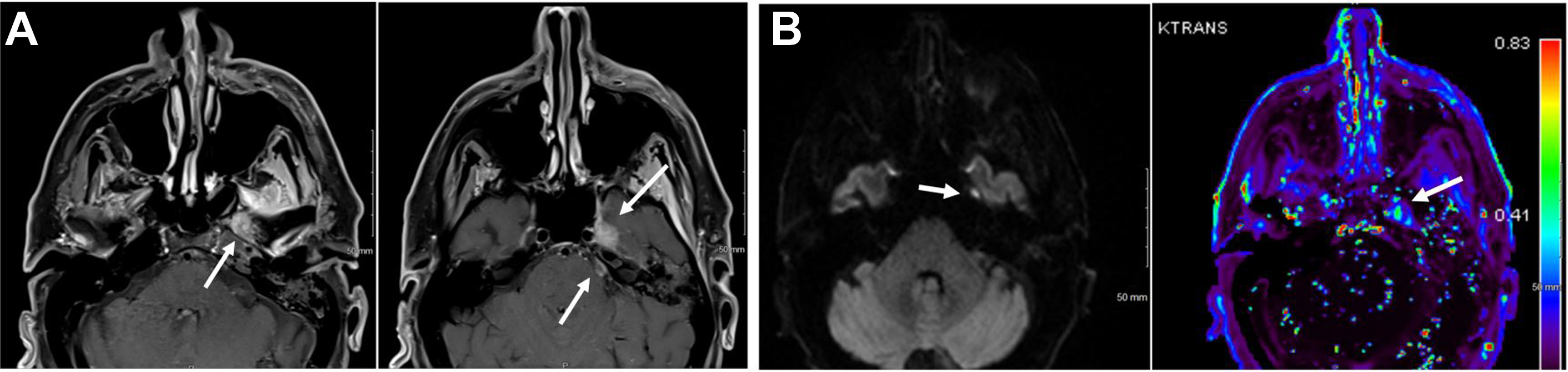
Perfusion Magnetic Resonance Imaging
Perfusion MRI is less frequently performed for the assessment of head and neck malignancies. The technique is based on the concept of interrogating a steady state of delivery of blood in normal tissues that can be disturbed by either increase or decrease in the vascular supply to the bed of tissues by using exogenous paramagnetic gadolinium chelates or by endogenous magnetically labeled arterial blood water acting as a diffusible tracer. The former technique is the basis for dynamic contrast-enhanced (DCE) perfusion studies and the latter technique forms the basis of arterial spin labeling (ASL) perfusion sequence.
DCE perfusion MRI permits the semiquantitative estimation of the vascularity of tissues depending on the flow intensity curves generated and measured after the bolus administration of contrast (see Table 2 ). Parameters such as maximum contrast index (CI), time to reach maximum CI, maximum slope, washout slope, and area under the curve (AUC) at a specific time can be measured. Pharmacokinetic modeling enables the measurement of parameters for the transfer of the contrast between the intravascular space to the extracellular extravascular space. Three of the most useful DCE parameters are Vp, Ve, and Ktrans. Vp is the volume of blood plasma, Ve is the volume of the extravascular extracellular space, and Ktrans is the rate of transfer from the blood plasma into the extravascular extracellular space. Ktrans essentially measures how leaky a system is, considering the flow, surface area, and permeability. Ktrans correlates with the wash in phase (the slope of signal gain) as gadolinium enters the tissue slab. An additional useful parameter is Kep, sometimes considered the opposite of Ktrans. Kep is the backflow leakage of material from the extravascular extracellular space into the blood plasma.
Application of DCE perfusion MRI in head and neck malignancies has been explored for the assessment of the prediction of tumor response ( Fig. 2 ), differentiating residual or recurrent cancers from posttreatment changes ( Figs. 3 and 4 ), and from differentiating squamous cell carcinomas from other head and neck malignancies which could influence treatment strategies.
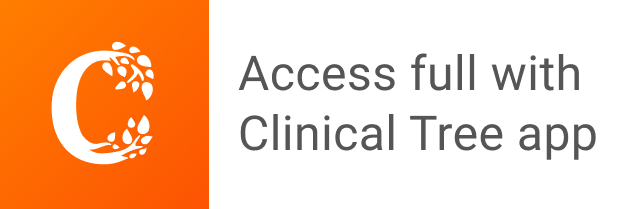