Advanced magnetic resonance imaging (MRI) techniques, such as magnetic resonance spectroscopy, diffusion MRI, and perfusion MRI, allow for a diverse range of multidimensional information regarding brain tumor physiology to be obtained in addition to the traditional anatomic images. Although it is well documented that MRI of rodent brain tumor models plays an important role in the basic research and drug discovery process of new brain tumor therapies, the role that animal models have played in translating these methodologies is rarely discussed in such articles. Even in consensus reports outlining the pathway to validation of these techniques, the use of animal models is given scant regard. This is despite that the use of rodent cancer models to test advanced MRI techniques predates and was integral to the development of clinical MRI. This article highlights just how integral preclinical imaging is to the discovery, development, and validation of advanced MRI techniques for imaging brain neoplasms.
Advanced magnetic resonance imaging (MRI) techniques, such as magnetic resonance spectroscopy (MRS), diffusion MRI, and perfusion MRI, allow for a diverse range of multiparametric information regarding brain tumor physiology to be obtained in addition to the traditional anatomic images. Although it is well documented that MRI of rodent brain tumor models plays an important role in the basic research and drug discovery process of new brain tumor therapies, the role that animal models have played in translating these methodologies is rarely discussed in such articles. Even in consensus reports outlining the pathway to validation of these techniques, the use of animal models is given scant regard. This is despite the use of rodent cancer models to test advanced MRI techniques predates and was integral to the development of clinical MRI. It is the aim of this article to highlight just how integral preclinical imaging is to the discovery, development, and validation of advanced MRI techniques for imaging brain neoplasms.
From almost the moment MRI was commercially available, its potential to become an indispensable tool central to the multidisciplinary planning of individualized brain tumor patient management was recognized. The inherent high resolution and exquisite soft tissue contrast of MRI allow radiologists, pathologists, neurosurgeons, neuro-oncologists, and radiation oncologists to gain an understanding of the 3-dimensional morphologic problem they face on a patient-by-patient basis. Paralleling this, many people in the research community (clinical and basic science) have been exploring the role that advanced MRI techniques may play in investigating the structural, functional, and metabolic nature of the brain tumor microenvironment. This exploration has been brought about by a desire of the clinical researchers and pharmaceutical companies to have access to early and noninvasive biological information that can predict outcome and/or quantify therapeutic efficacy. The most common and most developed techniques (although there are others) can be classified into 3 main categories:
- 1.
MRS for quantifying cell metabolites.
- 2.
Perfusion MRI for quantifying tissue hemodynamics (blood volume, flow, and vessel permeability).
- 3.
Diffusion MRI for quantifying tissue structure and microenvironment (cell density and white matter tractography).
These technologies are currently being investigated as biomarkers for early diagnosis, for predicting outcome in response to specific therapies, and to monitor therapeutic efficacy. The pathway to clinical and regulatory acceptance of MRI biomarkers is not entirely transparent. A biomarker needs to find a niche role in improving patient outcome and/or reducing costs in a clinical setting. For use in the drug discovery process, a biomarker needs to significantly improve a clinical trial of a new therapy by quantifying efficacy, aiding in patient selection, or helping with “go or no go” decisions. Demonstrating these abilities is not trivial and goes beyond clinical or scientific studies. However, what is necessary is that before a biomarker can be accepted as a surrogate marker, it must go through a process of validation and qualification through numerous scientific and clinical studies. In terms of validating biomarkers as surrogate endpoints in oncology research and drug discovery, it is necessary to establish strong scientific evidence of the biologic mechanism involved, acceptable analytical characteristics (sensitivity, specificity, reproducibility, and accuracy), and clinical feasibility. Just as new therapeutic agents must be shown to improve outcome of patients through regulated clinical trials, ultimately much of this validation must occur in the clinical setting by correlating biomarkers with clinical outcome for acceptance. This process is extremely expensive and time consuming, and it is often not ethical or possible to quantify image biomarker standardization and robustness through repeatability and dose-dependent experiments on only patients.
To this end, preclinical imaging of brain tumor animal models has played and will, for some time, play a vital role in the validation of numerous MRI biomarkers. This article shows by example how and why preclinical imaging is important to the validation of and to the fundamental understanding of each imaging biomarker. Although it is not possible to cover all potential brain tumor imaging biomarkers, it is hoped that by covering diffusion MRI, perfusion MRI, and MRS, it is possible to show the immense impact of preclinical imaging has had on the translation from biomarker concept to a clinically useful surrogate endpoint.
Magnetic resonance spectroscopy
In vivo MRS is an MR technique that allows for the detection of cellular metabolites whose protons have different MR frequencies from that of the surrounding water protons. The MRS data are acquired from either large single voxel localized by traditional MRI images ( Fig. 1 A, B) or from multiple voxels similar to traditional MR images. The data are usually presented in the form of a spectrum ( Fig. 1 C). Each peak represents the relative abundance of protons with different resonant frequencies caused by differences in their local magnetic field. The unique chemical structure of various metabolites results in different local magnetic fields experienced by their protons and results in a unique fingerprint-like MRS signature. Because MRS can be acquired from human and rodent tumors, it can be an excellent translational research tool or biomarker for quantification and imaging of tumor metabolism.
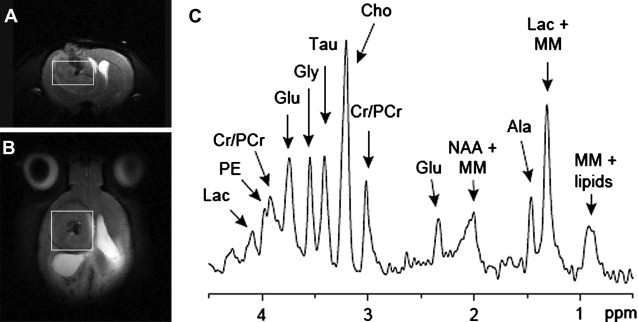
Investigation of brain tumor metabolism by MRS is one of the oldest clinical research applications of MR and predates the availability of clinical MRI scanners. Initially, phosphorous MRS was the most widely used technique, as it allowed for the quantification of high-energy phosphate metabolism as a biomarker of tumor hypoxia. However, since the introduction of clinical scanners, proton MRS has become the most popular MRS technique, as it allows for assessment of tumor metabolites using standard clinical MRI scanners and radio-frequency coils. Preceding the publication of the first clinical MRS results was an MRS study of the well-characterized C6 rat glioma model by Remy and colleagues. In this study the authors were able to resolve several different MRS resonance peaks, identifying 5 different metabolites: N -acetyl aspartate (NAA), lactate, lipid, choline (cho), and creatine (cr). Although it is now possible to quantify more than 10 important tumor metabolites (see Fig. 1 ) with modern MRI scanners, these original 5 MRS biomarkers are still the most commonly quantified. In addition to identifying these MRS peaks, this early study showed that the relative lactate, lipid, and cho signals increased, whereas NAA and cr decreased with increasing tumor burden. This established a link between MRS and tumor biology, showing that MRS had the potential to become an important noninvasive biomarker of tumor malignancy. Early clinical results showed that tumors had significantly different metabolic profiles compared with healthy brain tissue when measured by MRS. However, these significant differences between benign and malignant tumors were not universal. This prompted animal studies of various rodent brain tumor models to investigate the biologic phenomena that was being quantified by MRS. As a result of these studies, it was identified that MRS measures of tumor metabolism were extremely heterogeneous and brain tumors had overall lower metabolism compared with normal brain tissue contralateral to the tumor. This correlated with decreased tumor metabolism that was independently measured by bioluminescent quantification of tumor adenosine triphosphate, lactate, and glucose distributions. Paralleling the clinical results showed that the MRS tumor metabolic profile was unable to differentiate different types of tumor models or stage of development.
Despite the lack of specificity of MRS to predict tumor grade, these early animal experiments showed that MRS was still a potentially important biomarker because it could quantify tumor metabolic progression and/or therapeutically induced change in tumor metabolism. In a 9L gliosarcoma model, it was shown that MRS could reproducibly quantify decreased tumor metabolism associated with an efficacious cytotoxic agent.
Diffusion MRI
Diffusion MRI is an application of MRI that allows for the quantification and imaging of the random Brownian motion of water molecules within the cellular or tissue microenvironment. At first inspection, this may not seem like an important biophysical property that could help in the assessment of malignant brain tumors. However, it is emerging as an important imaging biomarker of therapeutic efficacy, of tumor invasion, and for tracking white matter fiber connectivity. The reason is that the cellular environment causes the restriction of this diffusion by, among other things, cell membranes, and so diffusion MRI can be used as a measure of cellular status and cytoacrchitecture.
Although diffusion-weighted MRI is often used clinically, the diffusion process can also be quantified by calculating the apparent diffusion coefficient (ADC), which when determined on a voxel-wise basis can generate a quantitative image. In such representations of the ADC, the membrane dense gray and white matter is hypointense compared with the cerebrospinal fluid. Analogous to MRS, it was the result of ADC measurements of rodent brain tumors that showed that diffusion MRI was a potential early biomarker of therapeutic efficacy. This change in ADC was then subsequently shown ( Fig. 2 ) to correlate with increased extracellular space and predict volumetric tumor shrinkage in a 9L gliosarcoma model receiving 1,3-bis(2-chloroethyl)-1-nitrosourea (BCNU, 13.3 mg/kg) treatment. In a thorough follow-up study, a dose-dependent assessment of ADC change was done in this rodent/chemotherapy model in parallel with clinical feasibility studies of the potential for ADC change to predict patient response to chemo or radiation therapy. These results showed empirically that ADC was negatively correlated with cell density and that ADC increased and cell density decreased significantly in a dose-dependent manner before changes in volumetric reduction in tumor size. Tumor progression after therapy caused by repopulating tumor cells also caused a substantial decrease in ADC before volumetric progression was measurable.
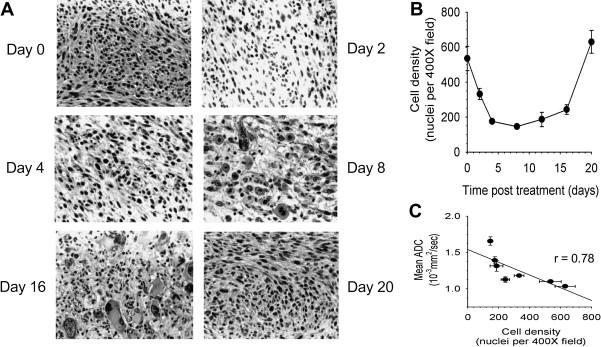
Although these initial results did prove promising, there were still several hurdles to overcome to clinically translate diffusion MRI as a biomarker for therapeutic efficacy. The heterogeneity of changes in ADC in human tumors compared with experimental rodent tumors was such that simple changes in mean ADC were not predictive of therapeutic efficacy and outcome. To overcome the inherent heterogeneity of changes in ADC in the clinical setting, the functional diffusion mapping (fDM) was developed as an alternative to mean ADC calculations. The calculation of fDM maps requires image registration of serial ADC maps acquired pretherapy and during chemo or radiation therapy followed by segmentation of the overlapping tumor mass into regions of positive, negative, and negligible change in ADC ( Fig. 3 ). Although the initial publication of fDM was applied to clinical cases and showed excellent correlation with patient outcome, it was impossible to prove that these regional changes in ADC actually predicted regional changes in cellular density. Thus, fDM imaging of rodent brain tumor models was important to show that fDM was reproducible, correlating linearly with survival and chemotherapeutic dose. The use of animal brain tumor models and sophisticated image registration techniques shows that these ADC changes also correlate with regional differences in cell density ( Fig. 4 ).
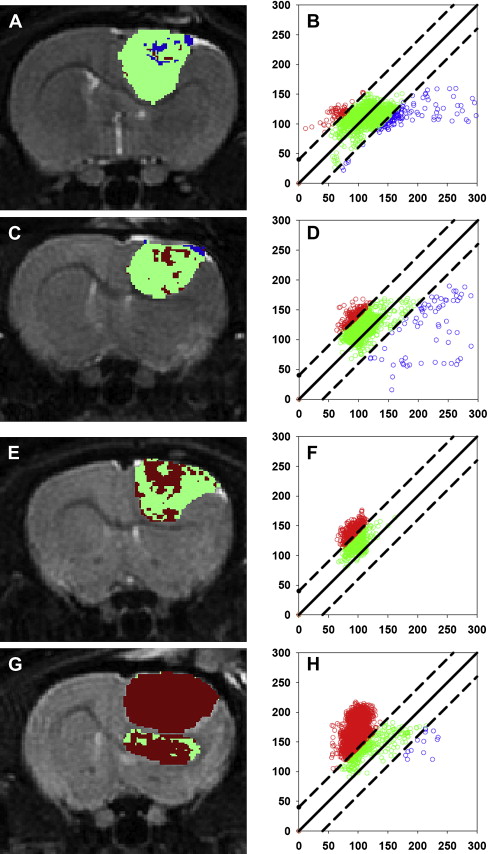
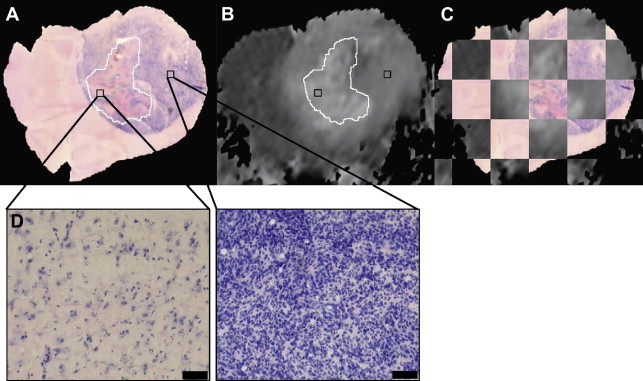
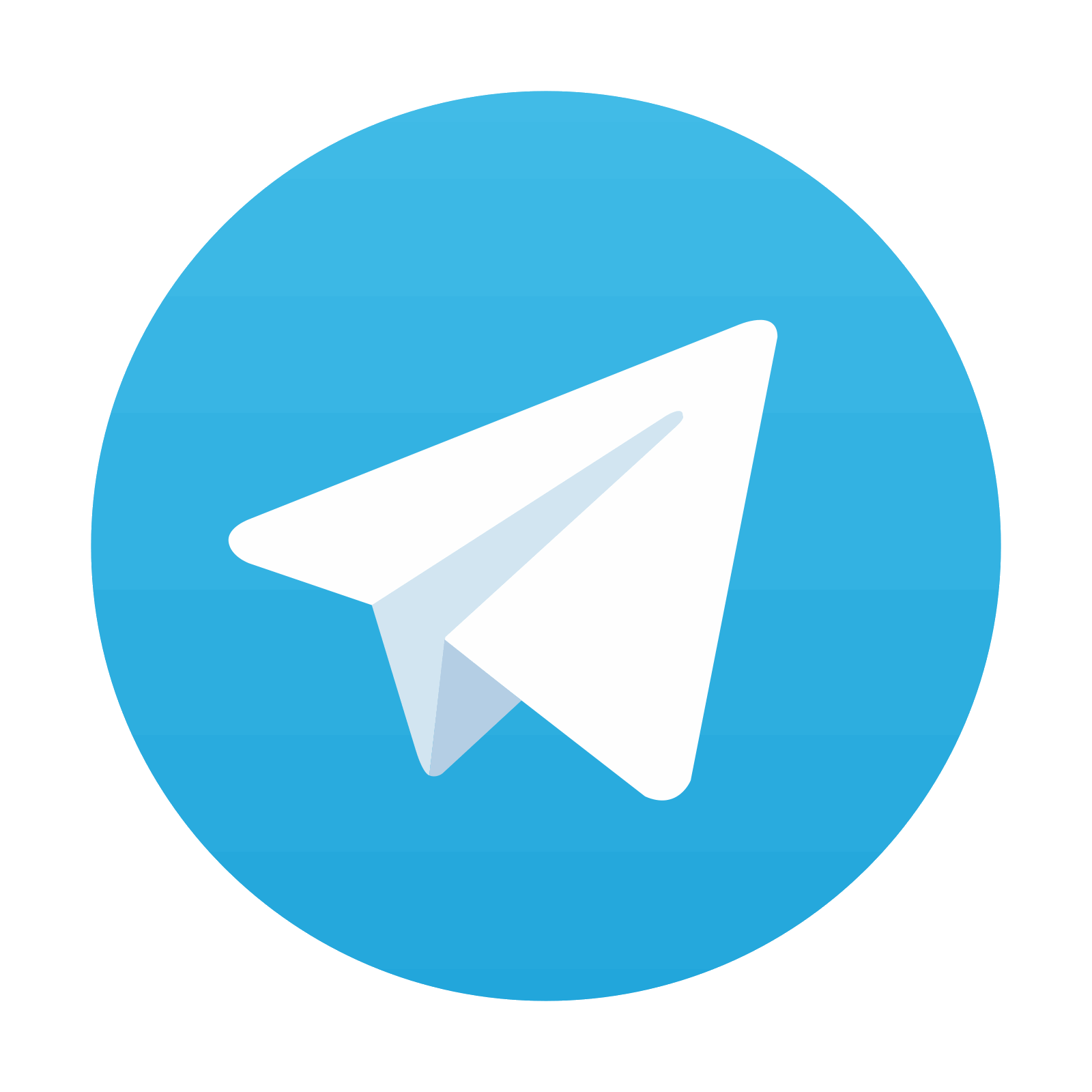
Stay updated, free articles. Join our Telegram channel
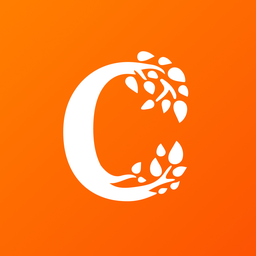
Full access? Get Clinical Tree
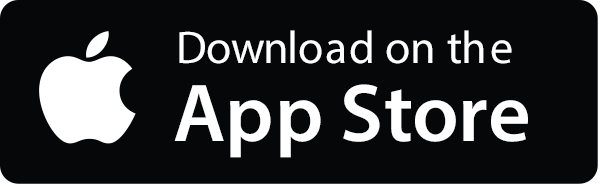
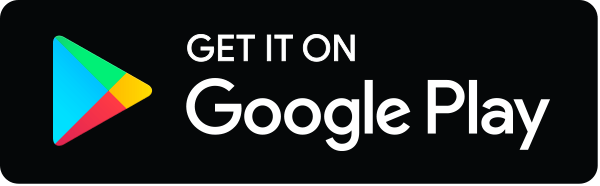
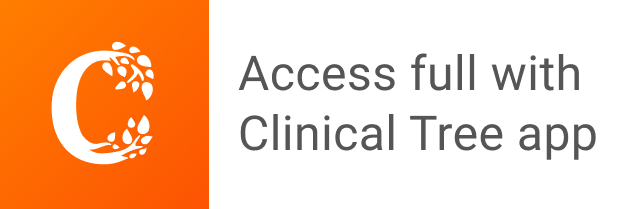