Fig. 1
The role of robots in surgery: a robotic device is an accurate, intelligent intermediary between the surgeon’s intent and action of the surgical instruments on the patient. Both telesurgical (master-slave) and directly manipulated (a surgeon’s assistant, where the slave subsystem is omitted) robotic paradigms have been used in augmented surgical procedures. For example, the former paradigm is used in widely used robotic surgery systems such as the da Vinci surgical system (Intuitive Surgical Inc.), and the latter in the MAKOplasty RIO orthopedic devices (MAKO Surgical Corporation, now part of Stryker). While visual feedback is always available to the surgeon in these procedures, neurosurgical and orthopedic robotic procedures typically include target guidance and navigation assistance integrated using a powerful computer workstation that uses intra-operative sensory feedback for registration of pre-operative and real-time imagery to provide augmented target visualization
For intra-operative guidance or robotic automation, accurate registration to anatomy is the most important procedure step, while safety and efficacy are the most important factors from the patient perspective.
In soft-tissue surgery, the lack of appropriate safety and efficacy evidence has hampered adoption in precision dependent specialties such as cardiology [2]. Similarly, neurosurgery has yet not widely used robotics [3].
By contrast, spine surgery provides a much closer approximation to other orthopedic surgery procedures (for example, the hip, knee, or the shoulder surgery) and the surgical goals and tolerances are relatively easily defined in geometric terms and robotic parameters. Recent wider acceptance of robotics among orthopedic surgeons has made some procedures both more commonly available as well as made robotics an invaluable tool. In their reviews of orthopedic robots, Mavrogenis et al. [4] present a clinical perspective on the adoption of orthopedic robots. Many reviews from engineering perspectives have also been published; for example, Sistona et al. [5] review the surgical navigation aspects for knee applications.
Orthopedic surgery performs fusing, shaping, or cutting of bones for either providing access or creating cavities for placement of implants. Many spinal surgery tasks, such as pedicle screws, spinal fusion, or disk implant placement are closely similar tasks from a robotics perspective to robotic orthopedic tasks. However, spinal surgery introduces unique challenges for the surgeon due to the complex three-dimensional anatomy, and placement of critical and delicate neural and vascular structure in close proximity to the bony anatomy being operated.
Conventional navigation mainly relies on identification of bone surface anatomy in 2D fluoroscopy images, providing robotic guidance and operation a great opportunity to improve safety, accuracy, as well as reduction in radiation exposure. Well-defined geometric accuracy goals [6] that prevent damage to the spine or peripheral nerves can be used for equivalent safety and efficacy evaluation. Large surgical volumes in procedures such as vertebroplasties, fusions, and biopsies and other spinal procedures [7, 8] strengthen the corresponding business cases for any devices.
1.1 Background
Robotics research in surgery is now several decades old (Fig. 2). The first widespread clinical robotic application was the ROBODOC system robot designed for automated milling of the hip cavity for implant placement in a hip replacement surgery [9]. This automation was particularly well received by users. While 10,000s of procedures were performed when the system was in active use, the lack of efficacy data for comparison with convention practice, and improvements in conventional treatment options, lack of FDA clearance, among other reasons withheld the system from reaching its full potential.
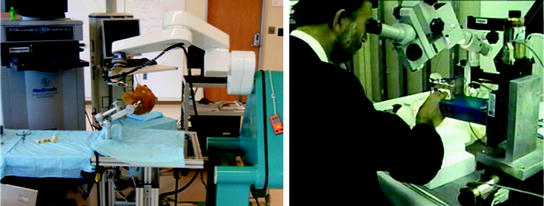
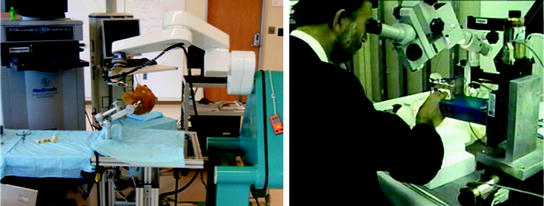
Fig. 2
Examples of research systems: a NEUROMATE (Integrated Surgical Systems, Inc.) neurosurgical experimental setup at the Johns Hopkins University (left), and a user manipulating the first generation “steady-hand” surgeon’s assistant microsurgery system (right)
A version of the system has recently received FDA clearance for milling of the implant cavity for hip surgery. ROBODOC Applications were also extended to include other joint reconstruction such as the knee, the domain of later more successful applications such as MAKOplasty. An integration workstation (Fig. 1) provided the registration and the user interface between the surgeon and the robot. The corresponding ORTHODOC planning software was designed to help a surgeon to graphically position a CAD model over a patient’s CT scan.
Registration determined the intra-operative spatial relationships between surgical instruments held by the robot, pre-operative CT imaging and the milling plan and the anatomy. Implanted pin fiducials, and later intra-operative surface digitization using a robot held digitizer was used for robot/CT/bone surface registration.
After initial manual preparation during surgery, this milling plan was executed automatically. After the milling, the surgery proceeded without the robot for the remaining portion. A contemporary robotic system for orthopedic surgery (the German CASPER system [10]) used a similar clinical workflow as the ROBODOC and was also not successful.
Similarly, the contemporary FDA-approved neurosurgery system (the NEUROMATE; Fig. 2, left) too was not widely applied clinically [11] and now exists primarily for use in further research and development. The reviews [12, 13] detail other early orthopedic robotic research applications. By the turn of the century, robotics research was receiving active attention for many other surgical specialties, such as eye surgery (the JHU “steady-hand” system [14]) that have also yet to be commercialized.
By comparison, led by successful robotic systems such as the AESOP (Computer Motion, Inc; now owned by Intuitive Surgical, Inc) camera assistants [15] for laparoscopic procedures (a modified SCARA architecture, with a passive remote center of motion), robots have achieved a much greater user acceptance. Other robotic surgery systems including the MIRO system [16] or the University of Washington RAVEN prototypes, and commercial complete general laparoscopic surgery robotic systems such as the da Vinci telerobotic system (Fig. 3) [17–19].
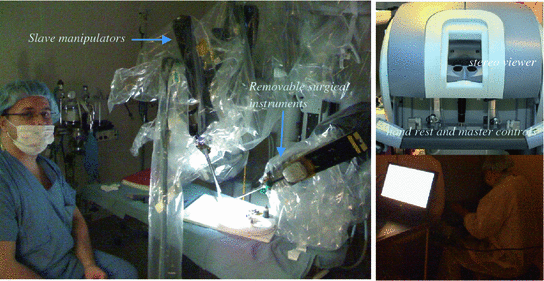
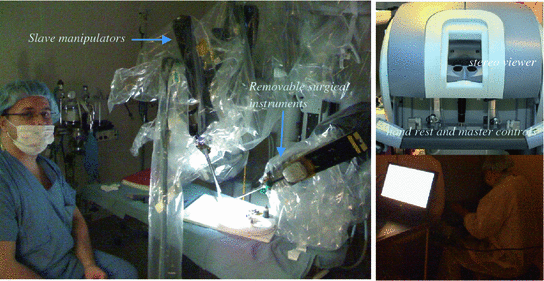
Fig. 3
The da Vinci surgical system (Intuitive Surgical, Inc.): a first-generation slave (patient-side manipulators) setup for a training session (left), and a second-generation master console (right, top) and the system in use by a trainee surgeon and bottom. Until the introduction of near infrared fluorescence integration recently, only visual imaging was available for feedback to the surgeon. Any additional imaging was viewable as a video (picture in picture) piped from external consoles (right bottom). A new version of the da Vinci system—the da Vinci Xi—has been introduced in 2014 that significantly improves positioning of the surgical instruments over the patient and reduces the operating room footprint
The da Vinci Surgical system (Fig. 3) remains the dominant commercially available telerobotic minimally invasive surgery system. This telerobotic system scales down the hand-motion depicting surgeon’s intent between the master manipulators and their configurable associated slaves. Laparoscopic robotic surgery with the da Vinci surgical systems is now widely used beyond prostate surgery. Active specialties include complex gynecological procedures [20], partial nephrectomies and other urological procedures [21]. Development and adoption for other procedures continues in cardiac surgery, head/neck surgery [22], and many other applications.
Now in the fourth generation (the da Vinci Xi), the da Vinci consists of several parts. A surgeon’s console contains the control handles (master manipulators) that are driven by the surgeon using laparoscopic instrument like grips, while viewing an auto-stereoscopic endoscopic view of the surgical site. A set of patient side manipulators hold the camera and the surgical instruments, and associated computing and stereo-endoscopic vision equipment completes the setup. With the instrument degrees of freedom included, the slave robots can be configured to have up to seven degrees of freedom in total.
A da Vinci system may mount up to four instruments, with one restricted to being the stereo endoscopic camera. The third-generation systems (the da Vinci Si) first allowed for up to two surgeon’ consoles [23] to be used simultaneously. A large catalog of 8 mm wristed rigid and 5 mm articulated (snake-like) removable flexible surgical instruments can be interactively mounted during surgery for specific surgical tasks (e.g. cutting, suturing, or cautery) as needed.
As noted by Shuford et al. [18], a majority of the approximately 75,000 radical prostatectomies performed in the United States annually were performed robotically by 2007, rising from only 18,000 procedures in 2005. Multiple large population and long-term studies show comparable or favorable performance of robotic methods [19] in urology.
1.2 Limitations of Current Systems
Current robotic systems suffer many limitations in addition to the substantial initial system cost, annual maintenance expenses, and the higher cost of the disposable surgical instruments compared to laparoscopy. Significantly long learning curves for clinical proficiency have also been reported.
These existing systems can’t be used in image-guided surgery as currently designed due to their size, limited accuracy, and interference with the conventional clinical workflow. Currently reported spine applications using the da Vinci systems are mostly forward-looking procedure development similar to other specialties such as head/neck surgery [22].
In such procedure development, intra-operative devices for registration have included instruments held by the robot, fiducials combined with C-Arm or other fluoroscopy, or optical and electromagnetic trackers such as the Axiem EM tracking (Medtronic Inc), or the Optotrak or Polaris systems (Northern Digital, Canada) systems. Intra-operative registration is performed similar to the spine navigation applications or the orthopedic surgery applications discussed above, typically by digitizing sufficient numbers of corresponding anatomical landmarks necessary for accurately computing a rigid registration.
The remaining sections of this article are limited to a narrow engineering perspective on robotic surgery developments specifically related to spine surgery. Non-robotic surgical navigation and registration are addressed separately elsewhere in this collection.
We also omit simulators for surgical training that may include robotic interfaces, robots for anatomical testing or rehabilitation, and external therapy or imaging robotic devices such as those for cone-beam computed tomography.
The reader is referred to clinical reviews for perspectives on human usage, for example, Bertelsen et al. [24] present a clinical perspective of spine related robotics and Rozer et al. [25] focus on the development and use of the commercial spine robots. For an even broader engineering review of robotic surgery the reader is referred to [26], or similar reviews [27, 28], and for clinical perspectives specifically on spinal robotics developments to reviews such as [8, 24, 25].
2 Recent Research and Developments
While clinical evaluations of robotic surgery often note [21] the need for additional information overlay as it is difficult for a human to interpret multiple sources of information presented together, it is also non-trivial to establish and maintain any registration in soft-tissue while it is being manipulated.
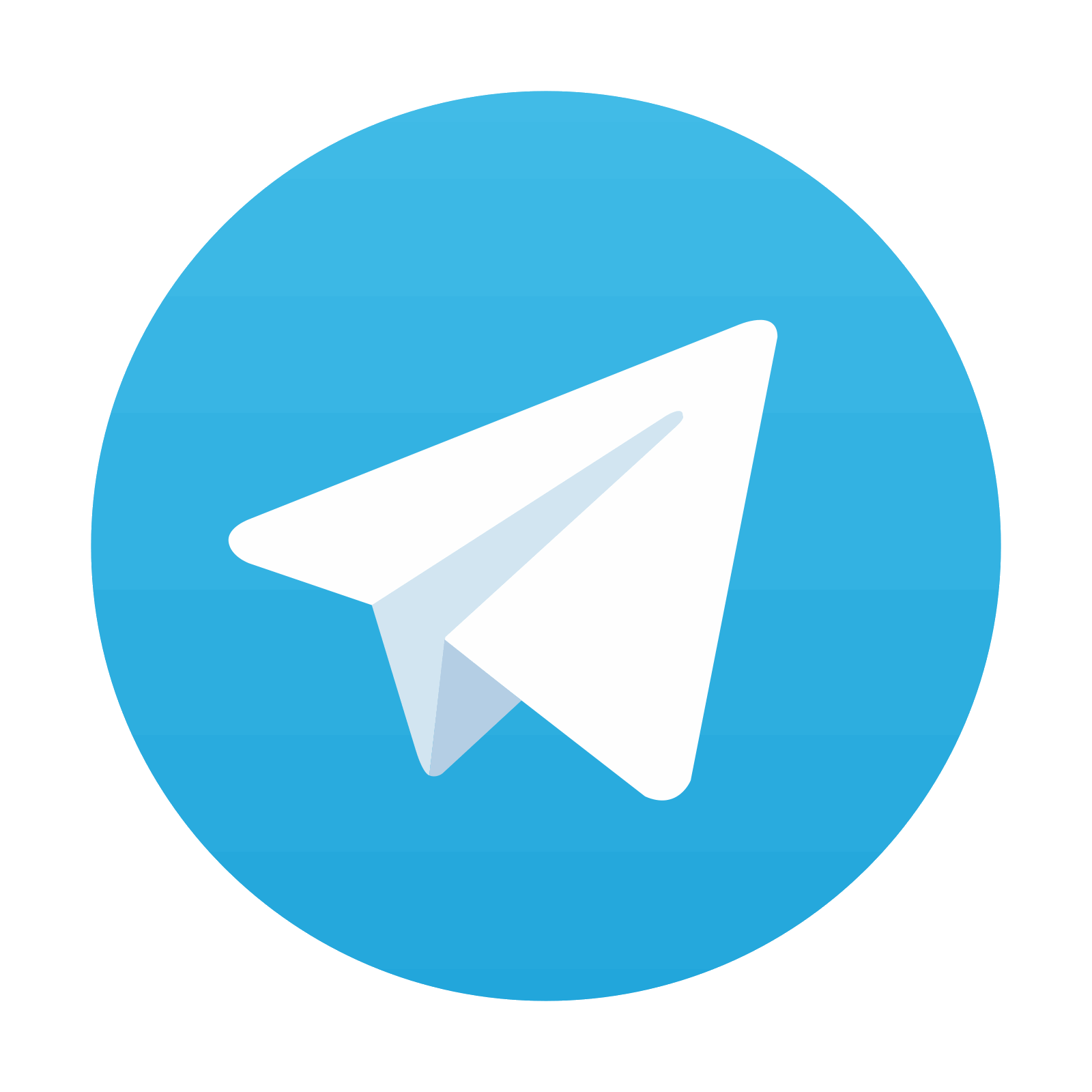
Stay updated, free articles. Join our Telegram channel
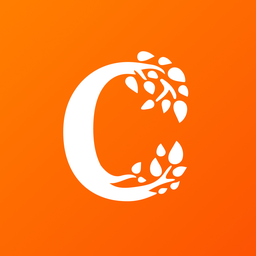
Full access? Get Clinical Tree
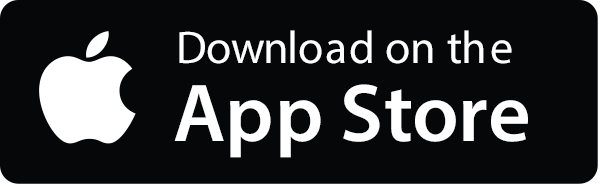
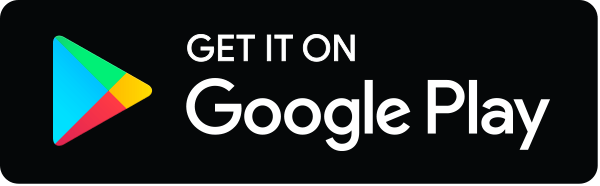