Radiation therapy is a major treatment modality for malignant and benign brain tumors. Concerns of radiation effects on the brain tissue and neurocognitive function and quality of life increase as survival of patients treated for brain tumors improves. In this article, the clinical and neurobehavioral symptoms and signs of radiation-induced brain injury, possible histopathology, and the potential of functional, metabolic, and molecular imaging as a biomarker for assessment and prediction of neurotoxicity after brain irradiation and imaging findings in radiation necrosis are discussed.
Radiation therapy (RT) is a major treatment modality for malignant and benign brain tumors. Concerns of radiation effects on the brain tissue and neurocognitive function and quality of life increase, however, because survival of the patients treated for brain tumors is improving. Radiation effects on the brain manifest as late neurologic sequelae and neurocognitive dysfunction with or without gross tissue necrosis. Late neurocognitive dysfunction presents as diminishing mental capacity for working memory, learning ability, executive function, and attention. Recent multicenter studies of patients with low-grade gliomas who are without clinical signs of tumor recurrence after radiation treatment show that both a high total dose and a high dose per fraction are associated with neurocognitive deterioration, especially memory functions. Radiation-induced functional, metabolic, and molecular changes in the brain structures and neural networks, which can be assessed by in vivo imaging, could be responsible for neurocognitive function changes.
This article discusses clinical and neurobehavioral symptoms and signs of radiation-induced brain injury; possible histopathology; and the potential of functional, metabolic, and molecular imaging as a biomarker for assessment and prediction of neurotoxicity after brain irradiation and imaging findings in radiation necrosis.
Clinical, radiologic, and neurobehavioral symptoms and signs
Clinical Symptoms and Signs
Classically, clinical complications after brain therapeutic irradiation have been described as acute (days to weeks after irradiation); subacute or early delayed (2–6 months after the completion of RT); and late effects (6 months to years after the completion of RT).
The acute reaction to conventional fractionated brain irradiation is usually mild, characterized by headache, nausea, drowsiness, and sometimes worsening of neurologic symptoms. Corticosteroids are usually successful in relieving acute complications.
Reports on early delayed reactions increase with frequency following contemporary cranial irradiation techniques. General neurologic deterioration during this interval (2–6 months after RT) is believed to be secondary to transient, diffuse demyelination. Many focal neurologic signs following radiation treatment of intracranial tumor have been attributed to intralesional reactions, probably indicative of tumor response or perilesional reactions (ie, edema or demyelination). Periventricular white matter (WM) lesions start to appear on conventional MR imaging or CT during this interval, however, even with standard fractionated partial brain RT. Following high-dose, volume-limited stereotactic radiosurgery (SRS), transient WM alterations are often apparent on conventional MR imaging, generally beginning 6 or more months after treatment. Following high-dose, large brain-volume treatment and concurrent chemotherapy, necrosis, particularly in WM, starts to develop in this interval, and the location of necrosis is often near the site of the original tumor.
The classical late effect following brain irradiation is either localized or multifocal necrosis, often associated with high-dose and large brain-volume treatment. Complications include worsening neurologic signs and symptoms, seizures, and increased intracranial pressure. Nevertheless, WM abnormality is a much more common late effect, and is often noted extending peripherally beyond the high-dose volume following partial brain irradiation. WM abnormality and necrosis are progressive and the imaging findings are discussed later.
Neurologic symptoms and neurocognitive impairments related to WM injury range from mild personality change to progressive memory loss, and to marked, incapacitating dementia.
Radiologic Signs
The radiologic signatures of WM alterations have been categorized as (1) periventricular changes, (2) focal extension of intense signal into WM, (3) diffuse extension into WM, and (4) diffuse coalescence of white and gray matter into intense signal region, loss of architecture, cortical atrophy, and hydrocephalus.
Following focal or whole-brain irradiation asymptomatic focal edema is a commonly seen finding both on CT and MR imaging, typically presenting as increased signal on T2-weighted and fluid attenuation inversion recovery images in the WM on MR imaging ( Fig. 1 ) and as decreased attenuation in the WM on CT.
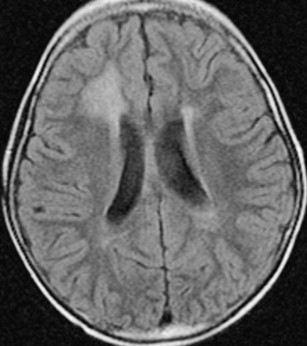
Radiation necrosis is often difficult to differentiate from recurrent tumor because the imaging pattern is very similar and they have many shared characteristics, such as an origin that often is at or in the vicinity of the original tumor, and they often demonstrate heterogeneous contrast enhancement. Commonly, radiation necrosis presents as a single focal enhancing lesion but it can be multifocal, or even in the contralateral size. The side may vary and range from small nodular enhancement to large areas of necrosis and heterogeneous enhancement. Most lesions consist of an enhancing mass with a central area of necrosis often in a so-called “soap-bubble” or “Swiss-cheese pattern” ( Fig. 2 ). On T2-weighted images, the solid portion of the radiation-induced necrotic mass has low signal intensity, and the central necrotic component shows increased signal intensity.
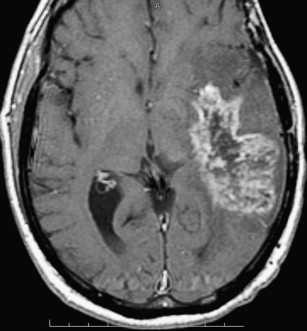
In the milder forms of radiation-induced injury the pattern of enhancement can be nodular, linear, or curvilinear and present as single or multiple lesions of varying sizes. Commonly, the lesion growth over time demonstrates surrounding edema, and causes mass effect. Typical locations for radiation necrosis are in the postsurgical tumor bed, in the periventricular WM especially corpus callosum and centrum semiovale (on top of the ventricles) because the periventricular WM is very susceptible to radiation. Radiation injury and radiation necrosis can occur outside the high-dose radiation dose field ( Fig. 3 ).
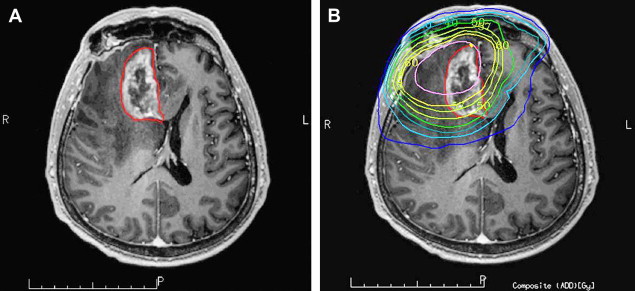
Neurobehavioral Symptoms and Signs
In recent years, many efforts have been focused on late neurocognitive dysfunction and quality of life of patients with brain tumors who had been treated by RT with or without concurrent chemotherapy. Although a few studies find that the deterioration of neurocognitive function is an indicator of tumor progression, a recent multicenter study of patients with low-grade gliomas who had no clinical signs of tumor recurrence at least 1 year after treatment showed that a high total dose correlated with a decline in working memory and that a high dose per fraction interfered with long-term memory storage and retrieval. Also, in a randomized trial of low- (50.4 Gy) versus high-dose (64.8 Gy) RT in patients with supratentorial low-grade glioma, significant cognitive deterioration from baseline was found in those without tumor progression, with rates of 8.2%, 4.6%, and 5.3% at years of 1, 2, and 5, respectively, as assessed by the relatively insensitive Folstein Mini-Mental State Examination. Moreover, the rate of cognitive impairment is even higher using a battery of neuropsychologic tests, which are much more sensitive to cognitive functions than the Mini-Mental State Examination. Also, neurocognitive dysfunction is observed without radiation necrosis, consistent with the findings in an animal study.
The cognitive domains of these dysfunctions present primarily in memory function, learning ability, and executive function, and to a lesser extent in fine motor skills and attention.
The potential effect of RT on neurocognitive outcomes is an important factor in the determination of the risks versus benefits of treatment, which should be an integral part of clinical decision-making. Given the late nature of neurocognitive dysfunction, it is important to identify in vivo imaging biomarkers for early assessment and prediction of late neurotoxicity.
Histopathology in radiation-induced brain injury
Radiation-induced injury in cerebral tissue is a highly complex and interactive process involving multiple tissue elements. Cerebral vascular injury has long been recognized to occur acutely and precedes subacute demyelination and reactive astrocytic and microglial responses. Histopathologic studies reveal that lifting of endothelium from the basement membrane, dilation and thickening of blood vessels, endothelial cell nuclear enlargement, and hypertrophy of perivascular astrocytes are among the first effects after irradiation. Early endothelial cell death and apoptosis after irradiation have been detected. Possible mechanisms of endothelial apoptosis include generation of intracellular ceramide by acidic sphingomyelinase and adhering leukocytes by tumor necrosis factor-α. The initial injury of vessels is followed by the formation of platelet matrix and thrombus, which eventually results in occlusion and thrombosis in microvessels within weeks to months. Furthermore, cerebral vascular injury is followed by degenerative structural changes in WM. The lag time between vascular injury and WM degeneration depends on the severity of the injury. Together, these observations strongly support the concept that cerebral vascular injury is of crucial importance for the development of WM injury following irradiation.
In addition to vascular abnormalities, demyelination is another typical histopathological of radiation-induced brain tissue injury. It has been shown that irradiation results in the loss of reproductive capacity of the oligodendrocyte type 2 astrocyte (O-2A) progenitor cells in both brain and spinal cord of adult rats. Presumably, radiation-induced loss of O-2A progenitor cells results in failure to replace normal turned-over oligodendrocytes, with the eventual consequence of demyelination. The kinetics of oligodendrocyte loss is inconsistent, however, with the late onset of necrosis.
The brain is a highly integrated system, comprising a number of disparate phenotypes of cells. Brain irradiation could affect not only vasculature and O-2A progenitors, but also astrocytes, microglia, neurons, and recently identified neural stem cells. As suggested, the response of neural tissue to irradiation also involves oxygen stress, inflammatory response, secondary reactive processes, and enhanced cytokine gene expression. To date, the understanding of histopathology and molecular biology after brain response to irradiation is limited.
Histopathology in radiation-induced brain injury
Radiation-induced injury in cerebral tissue is a highly complex and interactive process involving multiple tissue elements. Cerebral vascular injury has long been recognized to occur acutely and precedes subacute demyelination and reactive astrocytic and microglial responses. Histopathologic studies reveal that lifting of endothelium from the basement membrane, dilation and thickening of blood vessels, endothelial cell nuclear enlargement, and hypertrophy of perivascular astrocytes are among the first effects after irradiation. Early endothelial cell death and apoptosis after irradiation have been detected. Possible mechanisms of endothelial apoptosis include generation of intracellular ceramide by acidic sphingomyelinase and adhering leukocytes by tumor necrosis factor-α. The initial injury of vessels is followed by the formation of platelet matrix and thrombus, which eventually results in occlusion and thrombosis in microvessels within weeks to months. Furthermore, cerebral vascular injury is followed by degenerative structural changes in WM. The lag time between vascular injury and WM degeneration depends on the severity of the injury. Together, these observations strongly support the concept that cerebral vascular injury is of crucial importance for the development of WM injury following irradiation.
In addition to vascular abnormalities, demyelination is another typical histopathological of radiation-induced brain tissue injury. It has been shown that irradiation results in the loss of reproductive capacity of the oligodendrocyte type 2 astrocyte (O-2A) progenitor cells in both brain and spinal cord of adult rats. Presumably, radiation-induced loss of O-2A progenitor cells results in failure to replace normal turned-over oligodendrocytes, with the eventual consequence of demyelination. The kinetics of oligodendrocyte loss is inconsistent, however, with the late onset of necrosis.
The brain is a highly integrated system, comprising a number of disparate phenotypes of cells. Brain irradiation could affect not only vasculature and O-2A progenitors, but also astrocytes, microglia, neurons, and recently identified neural stem cells. As suggested, the response of neural tissue to irradiation also involves oxygen stress, inflammatory response, secondary reactive processes, and enhanced cytokine gene expression. To date, the understanding of histopathology and molecular biology after brain response to irradiation is limited.
Radiation necrosis and pseudoprogression
The differentiation of recurrent tumor or progressive tumor from radiation injury after radiotherapy is often a radiologic dilemma regardless of the technique used (CT or MR imaging). Most of these brain neoplasms have been subjected to radiation or chemotherapy and many of the tumors do not have specific imaging characteristics that enable the neuroradiologist to discriminate tumor recurrence from the inflammatory or necrotic change that can result from treatment with radiation or chemotherapy. Both entities typically demonstrate contrast enhancement. It is often the clinical course, a brain biopsy, or imaging over a lengthy follow-up interval that enable the distinction of recurrent tumor from a treatment-related lesion and not the specific imaging itself.
Although the difficulties in differentiation between radiation necrosis and a recurrent tumor often occur several months after the initial therapy, recent studies have described transient increases in contrast enhancement immediately after chemoradiation, which mimics tumor progression and has been termed pseudoprogression.
The incidence of pseudoprogression following concurrent chemoradiation has been reported to occur in approximately 15% to 30% of patients. Most patients remained clinically stable despite imaging changes suggestive of tumor progression. Radiation-induced vascular changes leading to focal transient increase in gadolinium enhancement following irradiation have been considered a possible mechanism. The combination of chemotherapy and RT may increase the incidence of pseudoprogression, possibly because of the increased radiosensitive effect of temozolomide on adjacent normal tissue. Pseudoprogression is further discussed elsewhere in this issue.
Imaging as a biomarker for radiation-induced neurotoxicity and radiation necrosis
Today, a large body of converging evidence from histopathology, molecular biology, animal models, and clinical observations suggests that radiation-induced neurotoxicity follows an interactive and dynamic sequence as early vascular injury, subsequent focal and diffuse demyelination, late tissue degeneration, and neurocognitive dysfunction. Although limited, functional and metabolic imaging have been used to investigate vascular injury, WM demyelination, and metabolic change in cerebral tissue after irradiation without apparent tissue necrosis. The functional and metabolic changes have been associated with radiation dose, dose volume, and fraction size. Furthermore, a few studies have attempted to link the functional and metabolic changes in the brain to neurocognitive function changes.
The following sections review the studies of WM injury and radiation necrosis using diffusion tensor imaging (DTI), and changes in cerebral blood flow (CBF), cerebral blood volume (CBV), and metabolism using functional MR imaging, proton spectroscopy, and positron emission tomography. Changes in CBF and CBV in the work-up to distinguish radiation necrosis from recurrent brain tumor are discussed elsewhere in this issue.
Diffusion Tensor Imaging
DTI is the most sensitive technique to assess WM integrity and histopathologic changes before structural changes are visible on any other imaging modalit. DTI is able to assess water diffusion and anisotropic diffusion in the tissue structures. In WM, the tight myelin sheaths surrounding the axon substantially restrict water diffusion in the direction perpendicular to the axon axis ( λ ⊥ ) compared with water diffusion in the direction along the axon axis ( λ | | ). Anisotropic water diffusion can be used to characterize tissue types (eg, grey and white matter), and to provide information on the density and orientation of WM fiber tracts. Furthermore, the quantitative indices obtained from DTI can aid in distinguishing between myelin loss and axonal injury. For example, an increase in λ ⊥ with or without a change in λ | | has been confirmed to be an in vivo biomarker for demyelination with pathology in myelin-deficient rats. In a recent study of radiation-induced WM damage in a rodent model, an early delayed increase in λ ⊥ after irradiation was correlated with demyelination histologically, whereas a decrease in λ | | was correlated with reactive astrogliosis without necrosis. Either λ ⊥ increase or λ | | decrease can lead to fractional anisotropy (FA) decreases.
DTI has been used to assess WM injury in pediatric and adult patients treated with brain radiation. In a recent study of children with medulloblastoma treated with craniospinal irradiation, decreased FA in WM after radiation was found to be correlated inversely with the age at treatment and positively with craniospinal dose. In a cross-sectional study of survivors of childhood medulloblastoma and acute lymphoblastic leukemia treated with craniospinal irradiation, differences of WM FA in the patients and in age-matched control group had a significant effect on intelligence quotient scores after adjusting effects of age at treatment, craniospinal dose, and time interval since treatment. In another study of the survivors of acute lymphoblastic leukemia 17 to 37 years after craniospinal irradiation, FA was analyzed in the temporal lobe, hippocampus, and thalamus, and found to be reduced compared with aged-matched control. Because neurocognitive functions in these patients were not evaluated, however, neurobehavioral consequences of degradation of these functional structures are unknown. Although findings from these cross-sectional studies identify several interesting factors that might contribute to radiation-induced neurocognitive injury in the pediatric population, future prospective studies are required to test hypotheses generated from these preliminary investigations.
In the adult patients who undergo partial or whole-brain RT, several prospective studies showed changes in DTI indices of normal-appearing WM. In a study of 25 patients who had high-grade glioma, low-grade glioma, or benign tumors and underwent partial brain RT, progressive decreases in FA from the start of RT to 45 weeks after were observed in large WM fibers of the genu and splenium of corpus callosum. Also, the decrease in FA was dose-dependent. Further analysis showed progressive increases in λ ⊥ but little change in λ | | , suggesting demyelination predominantly after WM irradiation. In another study of 26 patients who underwent prophylactic cranial irradiation, decreases in FA of several WM anatomic sites, including frontal WM, corona radiata, and cerebellum, were observed at the end of RT, and 6 weeks after RT, the extent of which seems to depend on risk factors of vascular diseases. Whether the radiation-induced WM injury is structural selective is a question that remains to be answered. Also, how these observed WM changes are associated with neurocognitive function changes remains to be tested.
Recent studies using diffusion weighted imaging to differentiate recurrent tumor from radiation injury have shown that the apparent diffusion coefficient (ADC) ratio in the contrast-enhancing lesion is lower in recurrent tumor than in radiation-induced injury ; however, other investigators using DTI demonstrated significantly higher ADC values in the contrast-enhancing part of the lesion in patients with tumor recurrence than in the contrast-enhancing lesion in patients with radiation injury. That study also showed that the ADC ratios in the WM tracts in the perilesion edema were significantly higher in radiation-injury patients compared with those with recurrent tumor and also that the FA ratios were significantly higher in normal-appearing WM tracts adjacent to the edema in patients diagnosed with radiation injury compared with those with recurrent tumours. Both λ ⊥ and λ | | values were significantly higher in contrast-enhancing lesions in patients with recurrent tumor than in those with radiation injury ( P = .02) and in the perilesional edema for both patient groups compared with normal-appearing WM. It can be anticipated that higher ADC values found in areas of tumor recurrence could be caused by increased extracellular space and micronecrosis, as commonly found in brain tumors, although a high-cell-density tumor exhibits low ADC. Lower ADC value in radiation injury could be a result of gliosis, fibrosis, macrophage invasion, vascular changes, and demyelination. These radiation-induced effects restrict water mobility (lower ADC) relative to simple noncellular or cystic necrosis, which elevates ADC. Contradictory are results from another study that demonstrated higher ADC values in treatment-related changes and radiation necrosis than in solid tumors, suggesting that solid tumors may have more densely packed cells than necrotic tissues, resulting in a lower ADC for recurrent tumor. It has been suggested and supported by data both from animal and human studies that diffusion imaging may be sensitive for evaluating early tumor response to therapy, suggesting that early increase in ADC values during therapy may relate to therapy-induced cell necrosis. The subsequent drop in tumor ADC to pretreatment levels could be an indicator of tumor regrowth.
Proton Spectroscopy
MR spectroscopy is a noninvasive technique for measurement of chemical substances (metabolites) in the brain and may serve as a sensitive imaging tool to noninvasively detect neurochemical changes as evidence of neurotoxicity in the irradiated brain. The technique has been used to differentiate recurrent tumor from radiation necrosis, whereas only a few prospective studies evaluating interval changes in metabolic activity in normal-appearing brain parenchyma during and following cranial RT for primary brain neoplasm have been published.
The most common technique has been single-voxel 1H MR spectroscopy technique with only a limited part of the brain evaluated and only at one or two time points during and after irradiation. Less frequently, two-dimensional multivoxel spectroscopy or three-dimensional spectroscopic imaging have been used for interval follow-up during or after RT.
It has been hypothesized that structural degradation in cerebral tissue after RT would be predicted by early changes in metabolic activity detectable by MR spectroscopy before the development of neurocognitive symptoms or anatomic changes seen on conventional MR imaging. This hypothesis is supported by the findings in a recent study of 11 adult patients with either low-grade glioma or benign tumors without previous cranial irradiation. That study demonstrated that significant alterations in brain metabolites occurred in normal-appearing human brain parenchyma early during radiation treatment and that interval progression of some of these changes occurred over at least a 6-month period. This was especially evident by the interval decrease in N -acetylaspartate/creatine (NAA/Cr) and choline/creatine (Cho/Cr) ratios from the pretreatment values at 3 weeks of radiation treatment and the progressive decline seen in the ratios at 6 months after the completion of radiation treatment ( Fig. 4 ). The conclusion from that study was that the decrease in the NAA/Cr ratio is most likely caused by neuronal damage, neuronal cell death caused by apoptosis, and neuronal dysfunction secondary to the irradiation. The metabolite NAA is predominantly present in neurons and believed to represent a marker of neuronal density and function, and creatine is a marker of energy metabolism and is considered to be fairly stable under most conditions. The presumption that NAA decreases following radiation is also supported by other previous studies demonstrating a decrease in whole-brain NAA and in the NAA concentration of irradiated brain. Observations of decreases in both choline and choline compounds, and the decreased Cho/Cr ratio, have also been reported after irradiation. The choline compound is correlated with cell membrane biosynthesis and metabolic turnover in proliferative tissue and it has been suggested that the decrease in Cho seen in normal-appearing brain tissue after irradiation might be caused by membrane damage in the myelin or the myelin-producing oligodendrocytes, accompanied by impaired tissue perfusion. One of the few previous reports of metabolic changes after prophylactic irradiation, in patients with acute lymphoblastic leukemia, found that the lower NAA/Cr and Cho/Cr ratio was associated with the presence of hemosiderin but not with imaging findings of leukoencephalopathy.
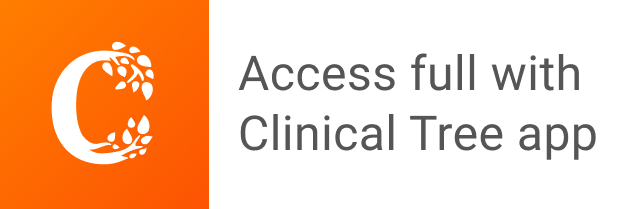