For decades, cardiovascular magnetic resonance imaging (CMR) and positron emission tomography (PET) have been clinically established imaging modalities in cardiovascular medicine. For several years, a new multimodality imaging system, PET/magnetic resonance (MR), using sequential or even integrated scanner platforms has been available. This hybrid imaging technique is gradually being implemented into the clinical setting for cardiac imaging.
Because of its unique capabilities, CMR has become a key imaging modality in clinical cardiology practice and a widely accepted standard of reference for the quantification of left and right ventricular function, the assessment of global and regional wall motion abnormalities and tissue characterization (scar, fibrosis, edema), as well as valve function. In contrast, PET is superb at quantification of myocardial perfusion and coronary flow reserve as well as visualization and quantification of particular metabolic processes at the molecular level. Combining both methods there is a range of complementary information, suggesting the use of integrated cardiac PET/MR may be justified in routine setting for evaluation of different disease entities. However, the exact role and value of PET/MR for cardiovascular imaging has not yet been determined. Critical evaluation of cardiac PET/MR is needed regarding incremental value beyond diagnostic information provided by PET and CMR alone. Because cardiac PET/MR is still in its infancy, this chapter will be based on published studies and case reports, available evidence, and where not yet available, on personal experience and expert opinion.
Technical Aspects and Implementation
Positron Emission Tomography/Magnetic Resonance Scanners and Instrumentation
To generate fused PET/MR images, several approaches exist. In the past the only practicable solution was to use software to register and fuse separately acquired PET and MR data. Although this method works relatively well for body areas with little deformability such as the head, imaging the heart poses problems with respect to coregistration as a result of patient breathing and cardiac motion, as well as patient positioning. Therefore sequential PET/MR systems provide improvement of coregistration if the patient undergoes both scans in a row on a mobile table system without repositioning. Compared with that, integrated PET/MR systems allow for a completely simultaneous data acquisition and in vivo observation of physiologic processes. Moreover, this technique minimizes the likelihood of movement-related misregistration and leads to a significant reduction of the scan time.
However, for PET/MR the technical problem of system integration is a major challenge attributed to the presence of magnetic fields. In PET/MR the real goal has been to fully integrate both systems without reducing the performance of the PET and the MR components. Uniform magnetic fields are of utmost importance in MR imaging. Thus any additional electronic circuits, which can distort the magnetic field, could potentially deteriorate the accuracy and quality of MR images. Besides the interference with the magnetic field, conventional PET photo-multiplier tubes (PMT) were not designed to be used inside strong electromagnetic fields and do not function properly in or near these fields. Consequently, the main challenge in combining PET and MR into one integrated system has been the development of MR-compatible PET detector technology. Current integrated PET/MR systems are either based on avalanche photodiodes (APD; Siemens mMR Biograph) or silicon photon multipliers (SiPM; GE Signa), where cross-interference with the MR is minimized.
Attenuation Correction in Positron Emission Tomography/Magnetic Resonance
For the attenuation correction of acquired PET data, modern PET and PET/CT systems use attenuation maps (µ-maps) that contain the radiodensity of each body volume element for 511-keV photons. These are typically calculated using transmission scans with external radionuclide sources or coregistered computed tomography (CT) data, which needs an additional transformation to convert to the radiodensity for 511-keV photons. However, for integrated PET/MR systems without a CT or external radionuclide source, new techniques for the creation of attenuation maps are needed. One approach is based on tissue segmentation using specialized computer algorithms to segment MR data into a fixed number of tissue types with a priori assigned coefficients of radiodensity. The currently most common approach uses a multipoint Dixon sequence for the segmentation into lung, fat, soft tissue, and background. However, one limitation of this method is that bones and calcifications are not assigned into a separate class but classified as soft tissue. Consequently, standardized uptake values of tissue close to bone might be significantly underestimated, which could particularly apply to the retrosternal parts of the heart. However, underestimation seems to be rather small in cardiac imaging.
To overcome this limitation, modified segmentation methods based on ultrashort echo time (UTE) sequences can be used, segmenting tissue with very short T2* (such as bone) into a separate class. However, due to a rather small field of view, this technique is not yet usable in cardiac PET/MR imaging.
Because of the fact that all objects between the patient and the PET detector can potentially attenuate and thus compromise the acquired PET data, all instrumentation used in the PET field of view during PET data acquisition has to be optimized for PET transparency. This has particular significance for radiofrequency surface coils, due to their unfavorable attenuation profiles. However, dedicated PET/MR surface coils with minimal attenuation for gamma quanta are commercially available. Alternatively, technical methods exist which allow for integration of attenuation coefficients of standard coil systems into the attenuation map after these have been previously measured in a CT scanner; however, one limitation of this approach is that the expansion of the attenuation map presumes knowledge of the exact coil position in the acquired image area.
Motion Correction
Electrocardiogram Gating
To assign image data to a specific cardiac phase, electrocardiogram (ECG)-based triggering is mandatory for MR and PET of the heart. However, during MR, and thus also during integrated PET/MR, ECG signals can be considerably distorted by the magnetic field and radiofrequency pulses. Therefore special care is needed when applying the electrodes and monitoring the signal. The comparably long cumulative acquisition times of most CMR protocols allow for an extensive parallel acquisition of PET signal, which typically compensates for the lost PET data because of ECG gating, resulting in reconstructed PET images of high quality.
Magnetic Resonance-Based Motion Correction
One of the most promising, yet still experimental, technical new developments of combined PET/MR is the advantage of motion detection using ultrafast (“real-time”) three-dimensional (3D) MR acquisition and tagging techniques. This allows for the MR-based estimation of motion-vector fields during PET acquisition, which can then be used to improve effective spatial resolution of PET and motion-induced inaccuracies in PET quantification. Recent advances in this area suggest a relevant utility of this technology for simultaneous PET/MR cardiac imaging.
Image Postprocessing, Visualization, and Quantification
To achieve a wide acceptance of complex cardiac imaging scans in clinical practice, a semiautomated or even automated processing of cardiac imaging data is required. Meanwhile, several software products are available from commercial and academic sources. However, most of these software products focus on either CMR or PET, but a few software packages analyzing both cardiac PET and MR data are available as well (e.g., Munich Heart or syngo.via). For analysis of clinical cardiac PET/MR scans, the respective software solutions should include tools for the assessment of ventricular function, viability, fibrosis and scar, perfusion, flow quantification, and tissue composition (e.g., T1, T2, T2* mapping) as well as dedicated postprocessing of cardiac PET data, including creation of bull’s eye plots and comparison of myocardial tracer uptake to normal databases.
Common Pitfalls
Despite its complexity, integrated cardiac PET/MR has been demonstrated to be a robust and reliable imaging modality. This is based on our own experience and hundreds of scans performed in several specialized cardiovascular imaging centers and research institutes around the world. Given a certain expertise and knowledge of common pitfalls, cardiac PET/MR has shown to be reliable and robust, and thus suitable for clinical routine imaging. Still, it is recommended that a team consisting of a radiologist and a nuclear medicine specialist with expertise in cardiac imaging should perform and interpret cardiac PET/MR, supervising technicians with cardiac imaging experience.
Segmentation and Misalignment Errors
The validity of attenuation-corrected PET data is directly dependent on the validity of the underlying MR tissue segmentation because the creation of µ-maps is based on the MR data segmentation. MR image artifacts or unexpected behavior of the segmentation algorithm can cause more or less severe tissue misclassification, compromising the validity of attenuation-corrected PET data caused by wrong attenuation-coefficients in the µ-map. Typical MR artifacts in cardiac imaging originate from foreign objects like implantable port systems, sternal wires, artificial heart valves, or artificial joint replacement of the humerus. Thus it is imperative that cardiac PET/MR reading should include visual inspection of the underlying µ-maps. If significant errors are evident, findings in attenuation-corrected PET data should be interpreted with caution and correlation with uncorrected PET data should be performed. If in doubt, an experienced reader should perform these tasks.
Another typical attenuation-correction (AC)–related issue results from patient motion. In a typical setting, the MR-based µ-maps are created in the beginning of the study and are later used for the AC of PET data that get continuously acquired over time in list mode. If the patient changes body position following µ-map creation, this results in misalignment between attenuation coefficients and PET data, which can cause severe PET image artifacts and quantification bias. As a simple workaround, it is recommended to perform repeated µ-map creation, interleaved between main MR acquisitions. If patient motion is retrospectively detected, PET list-mode data can be truncated to an interval before or after patient motion and an appropriate µ-map can be selected for attenuation correction.
Truncation of Field of View
Because of lack of space within the magnet and relatively long scan times, cardiac PET/MR is usually performed with the patient’s arms aligned along the body axis. Depending on the patient’s body habitus, this can result in parts of the arms being placed outside the MR field of view. This can cause so-called truncation artifacts at the edges of the attenuation maps, which can translate to errors in the attenuation-corrected PET data. These artifacts can at least partially be avoided by extension of the MR field of view by the use of optimized readout gradients or by partial correction with PET emission data using maximum likelihood reconstruction of attenuation and activity (MLAA). However, the MLAA algorithm is restricted to radiotracers that accumulate in the skin because it is based on contour detection in PET images.
Patient Preparation for Fludeoxyglucose Positron Emission Tomography/Magnetic Resonance Studies
Fludeoxyglucose (FDG) is still by far the most widely used tracer for cardiac PET. Normal myocardium is a very insulin-sensitive tissue that uses a variable mixture of glucose, lactate, ketone bodies, and free fatty acids under uncontrolled metabolic conditions, with a preference for fatty acids. Depending on the clinical question (inflammation/tumor vs. viability), myocardial metabolism needs to be shifted to either free fatty-acid or glucose utilization in cardiac PET with FDG. Therefore careful patient preparation is of utmost importance. It is strongly recommended to perform detailed patient interviews regarding the compliance to the preparation protocol. This is ideally performed by a nuclear medicine specialist before tracer injection. In cases of incompliance, the PET/MR scan can be postponed or certain countermeasures (e.g., insulin injection, unfractionated heparin injection, fatty-acid loading) can be taken. In addition to interviews, it is recommended to perform blood testing of glucose level and possibly insulin and free fatty-acid levels.
For cardiac FDG-PET of inflammation, infiltration, and tumors, suppress glucose uptake into normal cardiomyocytes by means of low insulin levels and high levels of fatty acids to differentiate between inflammatory infiltrates or tumor tissue and normal myocardium. This can be achieved using several techniques, including prolonged fasting, high-fat low-carbohydrate diet, fatty-acid loading, and additional injection of unfractionated heparin. However, fasting has been observed to be a major reason for patient discomfort, potentially contributing to increased cancellation rates during cardiac PET/MR scans. In a recent study, we have described a high-fat low-carbohydrate protein-permitted diet without fasting that yields an 84% success rate regarding suppression of normal myocardial glucose uptake. Cancellation rate was less than 3% and thus comparable with routine CMR scans.
For FDG-PET of myocardial viability it is necessary to significantly raise insulin levels to favor the uptake of glucose by normal cardiomyocytes. In principle, several techniques including hyperglycemic clamping, administration of hypolipidemic agents (e.g., Acipimox), and oral glucose loading are available. In our department, the following protocol for oral glucose loading has proved to be reliable, simple to perform, and well received by patients: patients fast before the scan, which normally means skipping breakfast in the morning. The glucose level of diabetic patients should be below 150 mg/dL (8.3 mmol/L). Before the FDG injection, the patients orally receive 75 g glucose in a preparation that is commercially available for glucose tolerance tests.
Radiation Exposure
The effective radiation dose for patients undergoing cardiac FDG-PET usually ranges between 4 and 7 mSv. Because the overall PET/MR study duration is determined by the comparably long CMR acquisition time, this allows for a significant prolongation of the PET acquisition interval, which might result in a significant reduction of the administered activity and therefore the effective dose. In our own institution the effective dose of cardiac FDG-PET/MR is approximately 2.5 ± 1.2 mSv, yielding still significantly better PET image quality than our standard cardiac FDG-PET/CT scans and leaving room for further reduction of the administered activity. Such radiation exposure goes significantly below that reported for 64-slice coronary CT angiography and competes with what is possible with third-generation dual-source CT.
Coronary Artery Disease
Suspected and Known Coronary Artery Disease
Published data on simultaneous PET/MR in patients with suspected or known coronary artery disease (CAD) is still sparse. The available data from PET/CT and MR studies indicate that simultaneous PET/MR has the potential to be a powerful tool for the detection of CAD and the assessment of myocardial viability. In integrated imaging protocols with PET tracers for perfusion imaging, viability imaging could be performed using late gadolinium enhancement (LGE) CMR, which seems not only to be the most sensitive modality for the detection of myocardial scar, especially for small subendocardial scars, but would also allow the omission of FDG imaging. This could greatly simplify the PET scan because the complex task of a dual tracer study would become obsolete. Moreover, myocardial perfusion PET has been demonstrated to achieve the highest diagnostic accuracy in noninvasive assessment of significant coronary artery stenosis and, in addition, allows for the absolute quantification of myocardial blood flow.
In side-by-side comparison using sequential FDG-PET/CT and LGE CMR in patients with known CAD, a close agreement between both modalities was found for the detection of transmural myocardial scars. However, a significant number of segments with subendocardial LGE showed normal FDG uptake by PET, thus indicating a higher sensitivity of LGE imaging for the detection of small myocardial scars. These findings have not yet been confirmed using simultaneous FDG-PET/MR.
Preliminary data show the feasibility of simultaneous PET/MR perfusion imaging using 13 N-ammonia. In this context, a protocol for the parallel acquisition and comparison of simultaneous PET and MR measurements of myocardial perfusion at rest and during pharmacologic stress has been developed. A more recent study compared 13 N-ammonia PET/MR with single-photon emission computed tomography (SPECT) perfusion imaging and reported superior specificity and diagnostic accuracy of PET/MR in patients with reversible ischemia.
Acute Coronary Syndrome
Infarct size is a strong predictor of outcome in patients with acute myocardial infarction (AMI). Both FDG-PET and LGE-CMR are clinically established techniques for the assessment of infarct size in the chronic state. Initial PET/MR studies in the subacute phase after AMI have also demonstrated moderate-to-good agreement between myocardial segments showing LGE and reduced FDG uptake ( Figs. 50.1 and 50.2 ). Furthermore, CMR in the subacute phase of reperfused AMI can differentiate between myocardial edema, microvascular obstruction, and intramyocardial hemorrhage, which is a potential indicator for reperfusion injury with additional prognostic impact also for the right ventricle.
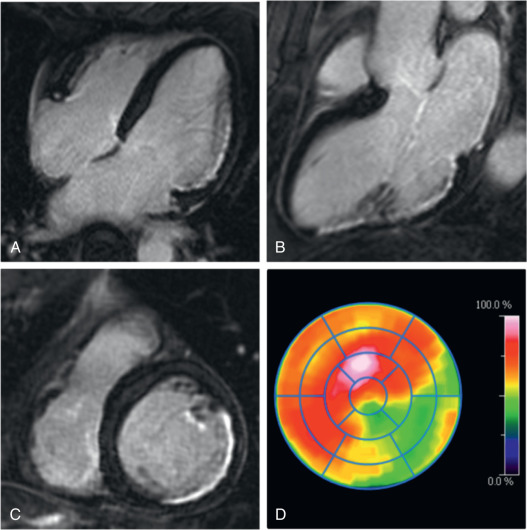
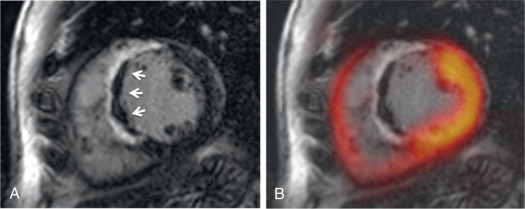
Other studies have observed a certain mismatch between PET and CMR. In cases of underestimation of infarct size using PET, this could be explained by the higher spatial resolution of CMR and a higher sensitivity to detect subendocardial infarction. However, recently performed studies have identified patients with AMI where some myocardial segments demonstrated reduced FDG uptake but no LGE ( Fig. 50.3 ). These myocardial segments had wall motion abnormalities comparable with that of infarcted (in terms of LGE) segments and showed only partial functional recovery after 6 months.
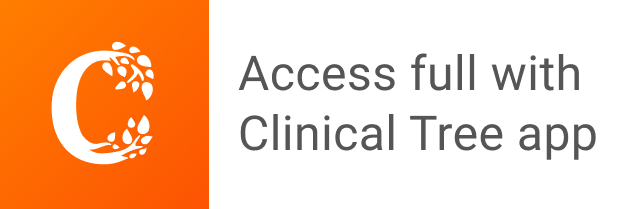