Magnetic resonance angiography (MRA) is an important imaging modality for the diagnosis, clinical workup, and treatment planning in patients suspected of a wide range of vascular pathology. The aim of MRA is to visualize the arterial and/or venous system by creating high contrast between the blood flow and its surrounding stationary tissue. To fulfill this aim, several techniques may be used in clinical practice. Depending on its demands and the conditions required to optimally visualize specific vessels of interest, imaging techniques are chosen. In the first part of this chapter, techniques will be addressed that are currently available and applied for MRA. Contrast-enhanced MRA (CE-MRA) is probably the most widely used MRA technique. CE-MRA is applied either by fast imaging of the first pass of an intravenously injected bolus of a gadolinium (Gd) chelate agent or by a prolonged imaging approach with a blood-pool contrast agent. The latter approach is applied when imaging requires a time window that surpasses the first arterial pass. Because of the reported adverse events, such as nephrogenic systemic fibrosis (NSF) (see Chapter 3 ), accumulation of Gd in the brain, kidneys, and bone tissue associated with Gd-based contrast agents, an increasing interest in non–CE-MRA techniques is currently observed. Black-blood and bright-blood non–CE-MRA techniques will be discussed. Finally, flow imaging by time-resolved three-dimensional (3D) phase contrast (i.e., four-dimensional [4D] flow magnetic resonance imaging [MRI]), with the aid of newly developed visualization tools, is a relatively new technique that may be used to visualize complex flow structures and add quantitative hemodynamic information.
In the second part of this chapter, we will address the anatomical regions imaged by MRA and discuss the state of the art. Special focus will be on the carotid arteries, thoracic and abdominal aorta, renal arteries, mesenteric artery, and the peripheral arteries.
Contrast-Enhanced Magnetic Resonance Angiography: Technical Approach
In CE-MRA, vessels in the field of view of interest are imaged during the arterial first pass of intravenously injected paramagnetic contrast material. These contrast agents have a short T1 relaxation time and will produce high signal on T1-weighted images. In clinical practice, Gd-bound chelates, for example diethylenetriamine pentaacetic acid (DTPA), are used as the paramagnetic contrast agents. The Gd ion is a rare-earth element, and toxic to humans when unbound. Therefore in MRA, Gd contrast is based on chelates to control the distribution of Gd within the body and to overcome toxicity while maintaining their contrast enhancement capacity. A very important property of these chelates is their chemical stability, which depends on the chemical structure and ionicity of the complex. A stable chelate has less tendency to release free Gd ions in the human body. Gd chelates can be distinguished in two structural categories: macrocyclic and linear Gd chelates. In macrocyclic structures, the Gd chelate is more stable and therefore the chance of free Gd ions in the body is reduced. Breaking of the bond between Gd and the chelate (transmetallation) is more likely to occur with linear agents than with macrocyclic agents. However, even though the macrocyclic agents are more resistant to transmetallation, development of NSF is described in patients with advanced renal failure (effective glomerular infiltration rate <20 mL/min/1.74 m 2 , acute deterioration of renal function or on dialysis) before Gd contrast administrations. Most reported cases of NSF fibrosis are linked with linear Gd-chelates. Awareness of this association has given rise to effective screening of patient with possible renal failure using the Choyke questionnaire, use of more stable Gd-chelates, and international recommendations for the use of Gd-based contrast agents in daily practice. Somewhat concerning are anecdotal reports of NSF-like symptoms following Gd administration but in the absence of renal dysfunction.
Gd-chelates create intravascular signal by shortening the T1 relaxation time of blood in proportion to the concentration of contrast material. At 1.5 T, T1 of blood is 1200 ms. The T1 relaxation time is shortened by Gd according to Eq. 44.1 :
1/T1=1/1200ms+R1[Gd]
Gd CE-MRA is insensitive to blood flow, in contrast to non-CE-MRA techniques as time-of-flight (TOF) and phase-contrast (PCA) MRA. Consequently, in CE-MRA, image quality is not degraded by flow disturbances.
Gd contrast agents can be classified depending on their distribution in tissue after intravenous administration, that is, extracellular or intravascular. Most of the Gd chelates used in clinical routine are extracellular agents. After intravascular administration they diffuse rapidly through the capillary walls into the extravascular space. In CE-MRA, arteries are preferably imaged during the initial passage of contrast. Timing of starting the image acquisition is essential to reduce signal from Gd diffused to the extracellular space and to overcome venous enhancement, which can lead to artifacts and image degradation.
The application of CE-MRA was improved by the introduction of Gd-chelates that have the capability to bind to larger molecules in the blood such as albumin and thus remain relatively intravascular. These contrast agents (e.g., gadobenate dimeglumine) provide a prolonged imaging time window because of a decreased decay time of contrast in blood. The use of such agents enables imaging beyond the initial passage of contrast bolus, which may be a benefit when acquisition needs to be gated to cardiac or respiratory motion, or when high resolution is required in small vessels such as the coronary arteries. When compared with an extravascular Gd-chelate, blood pool agents show an improved conspicuity of small vessels. Further development of macromolecular blood pool agents in the near future may play an important role in CE-MRA.
Three-Dimensional Contrast-Enhanced Magnetic Resonance Angiography Pulse Sequences
The CMR sequence used in CE-MRA needs to fulfill the following: T1-weighting is required, as well as the obtaining of a large 3D volume with sufficient spatial resolution, preferably fast within the first pass of contrast and within a breath-hold to suppress respiratory motion. Therefore fast T1-weighted 3D spoiled gradient-echo (GRE) sequences are used for image acquisition. In clinical routine, 3D CE-MRA preferentially is performed on a 1.5 T or 3 T system with strong gradient systems to reduce scan time, such that 3D datasets can be acquired during breath-holding. Typically, a short repetition time (TR) and echo time (TE) is used, 3 to 8 ms and 1 to 3 ms, respectively. Flip angle is 20 to 40 degrees to achieve optimized T1 weighting.
Furthermore, subsampling imaging techniques such as parallel imaging are implemented to reduce total scan time. Advanced k- space sampling techniques (spiral, keyhole, echo planar imaging [EPI]) may also be used to speed up acquisition.
At postprocessing, the 3D nature of the dataset allows viewing of the data from any desired angle, that is by creating multiplanar reformats (MPR) or rotational maximum intensity projection (MIP). This results in multiple images in various anatomic orientations. Thin (i.e., <3 mm thick) slices are required to provide a useful evaluation of these images. In some situations, it may be useful to use thicker slices (e.g., 10 mm) to reduce the number of slices and allow faster scanning. Although the ability to rotate the MIP is lost with such thick slices, scan times can be as short as 1 second.
Bolus Timing
Precise bolus timing is essential for first-pass CE-MRA. Arterial imaging is performed in the time window between arterial and venous enhancement and this time window depends on the rate of contrast agent injection, and will be patient specific. The transit time of contrast from the injection site (usually in the veins in the arm) to the field of view with the vessels of interest depends on several factors, for example, injection rate, injection site, heart rate, and stroke volume.
The Gd contrast concentration in the arterial blood is proportional to the injection rate and inversely proportional to the cardiac output:
[Gd]arterial=injection rate(mol/s)/cardiac output(L/s)
Maximum arterial signal intensity is achieved when the start of the acquisition and the contrast infusion are synchronized such that peak arterial Gd concentration coincides with the acquisition of the central portion of k- space. The center of k- space contributes most to overall image contrast, whereas the periphery of k- space provides image information of details and contributes to spatial resolution. Therefore for improved arterial/venous differentiation, central k- lines have to be sampled before venous return. In CE-MRA, different types of k- space filling can be used in the available 3D pulse sequences. It is important to adjust bolus timing to the order of k- space filling used.
In linear ordering, lines of k- space can be acquired in any order (high k- space lines first, low k- space lines first, or at random). Linear ordering can be used in a CE-MRA protocol when precise timing of the contrast bolus is difficult or arrival time may be prolonged.
For most CE-MRA examinations, elliptical-centric ordering is the preferred k- space sampling method used. Typically, the center of k- space is acquired first and the periphery later (e.g., elliptic-centric Contrast-ENhanced Timing Robust Angiography [CENTRA], Differential Rate K- space Sampling [DRKS], or PEak Arterial K- Space filling [PEAKS]).
To determine the delay between the start of the venous contrast injection and the start of the acquisition, either a small test bolus or fluoroscopic real-time imaging can be used. The timing method by using a test bolus (1 to 2 mL) is robust and easy to perform. However, it will lengthen the procedure by a few minutes and requires an additional administration of contrast. In fluoroscopic real-time imaging, the inflow of contrast is imaged in the field of view with the vessels of interest. At the moment of contrast arrival, the acquisition is started automatically; however, the short delay of a few seconds, which is needed to switch from the fluoroscopic real-time imaging to the actual start of CE-MRA acquisition, can be a disadvantage.
Contrast-Enhanced Versus Non–Contrast-Enhanced Magnetic Resonance Angiography
The association between CE-MRA and NSF in patients with severe renal dysfunction and linear Gd-chelates has been appreciated for over a decade.
Moreover, Gd accumulation in tissue in patients without renal impairment has been reported in several studies. Kanda et al. reported residual Gd concentrations in the brain, particularly in the dentate nucleus and globus pallidus, of patients without severe renal dysfunction and foci of hyperintensity on unenhanced T1-weighted magnetic resonance (MR) images associated with previous administration of linear Gd-chelates, and which may be associated with the total number of previous Gd-based contrast material administrations. Deposition of Gd has also been demonstrated in bone, skin, and liver. Currently, previous administration of macrocyclic Gd-chelates shows no association with Gd accumulation in tissue ; however, these macrocyclic contrast chelates have been in use in clinical practice for a shorter time than the linear chelates. Nevertheless, the application of CE-MRA and the amount of administered Gd contrast is of clinical importance, especially in patients with impaired renal function, and issues of long-term retention may be of particular concern in children/young adults. Awareness of NSF-related incidents associated with CE-MRA and reports on accumulation of Gd in various tissues have also led to a renewed interest in non–CE-MRA. Improvements in CMR hardware and software, including the widespread availability of parallel imaging have helped to reduce acquisition times and have made some non–CE-MRA methods clinically practical. Non–CE-MRA methods may be classified into three categories: black-blood, bright-blood, and 4D flow visualization.
Black-Blood Imaging
Black-blood imaging of blood vessels uses double-inversion recovery to null the signal of flowing blood. This technique is flow-sensitive. Two 180 degree inversion recovery prepulses are applied to null the signal of flowing blood: the first prepulse is nonslice selective and inverts the longitudinal magnetization vector in the entire body, whereas the second inversion prepulse is slice selective and inverts the magnetization in the imaging slice back to its original orientation. Signal of blood entering the imaging slice after the second prepulse has undergone reinversion and will appear dark in the images. Electrocardiogram (ECG)-gated partial Fourier fast spin echo (FSE) is the sequence of choice, using a slice thickness of 6 to 8 mm stacked in the transverse plane of the chest or in a double-oblique sagittal view of the aorta (i.e., the candy-cane view). Single-shot FSE (SSFSE) sequences are much faster than basic FSE sequences and allow for acquisition of the entire imaging stack in a single breath-hold. Fat saturation is recommended to increase the conspicuity of aortic wall hematoma. Despite being an established technique, black-blood imaging is prone to artifacts because inadequate nulling of blood signal may occur by slow flow, or when in-plane blood flow is present.
Bright-Blood Imaging: Time of Flight
TOF methods depend on the inflow enhancement of flowing blood and the relative saturation of longitudinal magnetization of stationary tissue to produce high contrast. The longitudinal magnetization from the background tissue is suppressed by multiple radiofrequency (RF) excitations, such that the magnetic spins associated with background tissue do not have sufficient time to fully regain their longitudinal magnetization. Saturation of background signal will reach a steady state determined by the flip angle, repetition time, and the T1 of the background tissue. This saturation will make stationary tissue appear dark in TOF images. The bright signal intensity of flowing blood is the consequence of the continuous inflow of fresh, unsaturated blood into the imaging slice, which produces more signal than the surrounding stationary tissue because the latter was repeatedly exposed to RF pulses. This effect is known as flow-related enhancement and can be maximized by using sequences with a short TR. Ideally, the imaging slice should be thin and oriented perpendicular to the direction of flow. ECG gating can be used to optimize synchronization of acquisition to the blood flow to the cardiac cycle and consequently to the signal present in the artery of interest.
Two-dimensional (2D) and 3D TOF techniques are in use. Two-dimensional TOF with consecutive slices stacked together can produce excellent contrast in the vessels when the imaging slices are planned perpendicular to the vessels of interest. When in-plane flow occurs, the longitudinal magnetization of blood will become saturated and signal is progressively lost. Additionally, venous signal may be suppressed with presaturation slabs, positioned parallel and next to the imaging slices, to saturate longitudinal magnetization of blood flowing into the imaging slice from the opposite side of arterial blood flow. Resolution of 2D TOF is often limited by the slice thickness used. However, thin slice acquisitions are possible, but acquisition time will increase with the number of slices required to cover the vasculature and signal-to-noise ratio (SNR) will decrease with decreasing slice thickness.
Three-dimensional TOF may be performed with isotropic voxel sizes of 1 mm 3 . High resolution is essential to visualize small branch vessels, stenosis, or to provide a clear delineation of tortuous vessels. Three-dimensional TOF has to deal with saturation effects of arterial blood flow, especially slow flow. On the other hand, the SNR will be increased by using 3D imaging. Decreasing the flip angle may reduce excessive saturation effects.
Bright-Blood Imaging: Phase-Contrast Angiography
In PCA, the contrast between flowing blood and the surrounding stationary tissue is generated by the flow-induced phase shift in the transverse magnetization of the moving blood. As the magnetic field strength and the precession frequency of spins are linearly related, the precession frequency will increase when spins move along a positive gradient in the magnetic field. Compared with spins that remain fixed in position (i.e., in stationary tissue), moving spins (i.e., in flowing blood) will accumulate phase in the transverse magnetization. This phase shift is proportional to its velocity and the gradient strength. In addition to such a velocity-sensitive acquisition, a velocity-compensation acquisition is defined. Such acquisition uses a bipolar gradient with equal positive and negative effect to achieve no difference in net phase shift between spins moving with uniform velocity. PCA uses the subtraction of both velocity-sensitive and velocity-compensation acquisitions to visualize moving spins. The velocity can be directly quantified from the resultant phase images. A priori to the acquisition the velocity sensitivity needs to be determined by choosing the Venc (velocity-encoding range), that is, the outer limit of the velocity range that is encoded by the phase shift of +180 degrees and −180 degrees.
TOF and PCA, two conventional bright-blood non–CE-MRA techniques, were highly time consuming and moreover, suffered from artifacts caused by turbulence and in-plane saturation. In a metaanalysis, the sensitivity and specificity for the detection of significant stenosis (>50% luminal reduction) for 2D TOF ranged from 64% to 100% and 68% to 96%, compared with 92% to 100% and 91% to 99%, respectively, for CE-MRA. Such limitations resulted in declining interest for TOF and PCA in favor of CE-MRA. However, in association with the reported adverse events from Gd usage, an increase in interest in new bright-blood non–CE-MRA techniques is occurring.
Bright-Blood Imaging: Steady-State Free Precession
Steady-state free precession (SSFP) produces high signal intensity for blood, because of the inherently T2/T1-weighted image contrast, which is high for all fluids, independent from inflow effects. ECG-gated cine acquisitions with balanced SSFP (bSSFP) are used to visualize the anatomy of thoracic aorta with breath-hold sequences. Aneurysms, coarctations, stenoses, and dissection flaps can be visualized using this approach. The aortic root is best evaluated in multiple planes, including long-axis planes of the left ventricular outflow tract (LVOT). The remainder of the thoracic aorta is best evaluated with images in the axial plane of the chest and in the double-oblique sagittal “candy-cane” view. bSSFP sequences are susceptible to magnetic field inhomogeneity, which are particularly present at high field strength. Off-resonance artifacts may result in nondiagnostic images. Dedicated shimming algorithms may aid in minimizing these off-resonance artifacts.
Bright-Blood Imaging: Dixon
Another relatively new bright-blood approach relies on an old CMR technique based on the chemical shift between both water and fat. Dixon’s water–fat separation uses the phase shift from the water–fat resonance frequency and, by using carefully chosen multiple echo times, excellent separation between water and fat is achieved in four distinct images: separate water and fat images as well as images with signal from both tissues in and out of phase. The Dixon technique may be applied for noncontrast MRA purposes and its application may be promising for imaging of the coronaries as well as the thoracic aorta.
Four-Dimensional Flow Visualization
Phase-contrast imaging enables quantitation of blood flow velocity in the direction of a magnetic field gradient. In addition to conventional through-plane one-directional velocity-encoding, advanced 3D time-resolved imaging is possible with velocity-encoding in all three directions, providing the temporal distribution of a velocity vector field, representing the flow of blood inside a 3D volume (i.e., so-called 4D flow data). Visualization tools such as streamline and pathline visualization have been developed to display this data and to segment blood vessels of interest from their surrounding tissue. The usability of this technique has been shown in the aorta, pulmonary arteries, carotid artery, and abdominal circulation as well as the circulation in the brain. Although this technique is still a research application, a lot of effort has been made to translate this technique toward clinical use. The additional hemodynamic information (e.g., wall shear stress, stenotic pressure drop, pulse wave velocity, viscous energy dissipation) which becomes available with 4D flow CMR in combination with the excellent 3D visualization possibilities of complex vascular structures and pathologies, may eventually push this modality to be a clinically viable application.
Artifacts in Contrast-Enhanced Magnetic Resonance Angiography
In CE-MRA, images with maximum arterial signal intensity are obtained when the acquisition of the central portion of k- space coincides with peak arterial Gd concentration. Timing of bolus arrival and imaging within the time window of arterial enhancement is critical to minimize artifacts. Starting the acquisition too early, when the contrast bolus is still arriving in the vessels of interest, can cause ringing or so-called Maki artifacts. Starting the acquisition too late will lead to imaging outside the time window of arterial enhancement, causing venous enhancement hampering the evaluation of arterial vasculature. Such artifacts may result in degradation of image quality and misclassified diagnosis.
Artifacts are most evident when the center of k- space is acquired before the peak of intravascular Gd arrival. The Maki artifact, for example, results in a central dark line in the vessel, from which a so-called pseudodissection can appear, when the contrast bolus arrives in the vessel of interest after sampling of the central k- lines. Overestimation of the length of a stenosis can occur due to signal loss because of turbulence and in-plane saturation. Generally, this latter artifact is not present in CE-MRA; however, signal loss can occur in the stenosis caused by high velocity rates, especially in combination with low contrast material concentration.
Erroneously, an occlusion can be suggested in tortuous arteries when the vessel of interest is not completely covered inside the field of view. Parallel to vessels with very high signal intensity, ghosts (i.e., phase artifact) can be seen.
Movement of the patient between the acquisition of the mask and the CE-MRA may lead to subtraction artifacts, leading to image degradation. Signal loss caused by susceptibility can be seen when clips, metallic stents or prostheses are present.
In non–CE-MRA, artifacts may also result in image degradation or even lead to misdiagnosis. Poststenotic signal loss due to turbulence or in-plane saturation in phase-contrast imaging may lead to an overestimation of a stenosis. When the a priori set velocity encoding (Venc) sensitivity is set too low, aliasing will then occur in the velocity images and when uncorrected will result in a decrease in signal in the phase-contrast magnitude images.
On the other hand, slow flow in time-of-flight CMR imaging may lead to similar misdiagnosis when vascular signal decreases. In black-blood imaging, in-plane flow may lead to vascular enhancement and inadequate signal suppression when the imaging slice is too thick.
bSSFP is very susceptible to off-resonance artifacts, especially at higher field strength and nonoptimal shimming, which may degrade image quality to the point of nonevaluable for clinical diagnosis.
Magnetic Resonance Angiography of Extracranial Carotid Arteries
Atherosclerosis of the extracranial carotid arteries is an important cause of stroke. Worldwide stroke is a major health concern, with nearly 800,000 strokes in the United States every year, including ~610,000 first-stroke patients and 185,000 patients with recurrent stroke. Stroke can lead to significant disability in nonfatal cases and accounts for approximately 1 of every 20 deaths in the United States.
Carotid atherosclerosis is a significant factor for risk stratification and is associated with more than a doubling in the stroke risk after a transient ischemic attack (TIA). Therefore accurate evaluation of the extracranial carotid artery is essential.
For evaluating the severity of carotid stenosis, x-ray digital subtraction angiography (DSA) remains the standard of reference. This imaging approach has certain limitations, including invasiveness and the use of ionizing radiation and iodinated contrast. Moreover, DSA involves a small risk of stroke, estimated at 0.5% to 4%.
Therefore, today, noninvasive imaging techniques play an important role in the routine assessment of patients with suspected carotid artery. Noninvasive techniques such as duplex ultrasound (DUS), CE-MRA and computed tomography angiography (CTA) are widely available and have largely replaced invasive x-ray arteriography in daily clinical routine.
When compared with DSA, noninvasive imaging techniques generally are less accurate for low-degree carotid artery stenoses. Metaanalysis showed sensitivity and specificity for DUS of 36% and 91%, respectively, 50% to 69% for stenosis degree of the internal carotid artery, 77% and 97%, respectively, for CE-MRA, and 67% and 79%, respectively, for CTA. Noninvasive techniques revealed higher sensitivity and specificity for high grade stenosis (70%–99%), DUS 89% and 84%, CE-MRA 94% and 93%, and CTA 77% and 95%, respectively.
DUS is widely available, easy accessible, cost effective and often used as a first-line investigation in standardized protocols assessing carotid artery disease. In experienced hands it is an accurate test; however, it has higher interobserver variability and lower sensitivity and specificity when compared with CE-MRA and CTA. Guidelines of the American Heart Association (AHA) and the American College of Cardiology Foundation (ACCF) recommend evaluation of the carotid artery by MRA or CTA, when DUS provides equivocal or nondiagnostic results. When DUS detects a significant stenosis (>70%) it is useful to evaluate stenosis severity by performing MRA or CTA. For assessing carotid artery stenosis, CE-MRA shows a comparable accuracy.
However, CTA has important limitations. It employs potentially harmful ionizing radiation and the administration of larger volume of iodinated contrast with possible toxicity and adverse reaction. Moreover, plaque calcification may hamper stenosis gradation. Therefore it is becoming common practice to combine DUS as first-line screening modality followed by CE-MRA for gradation of carotid stenosis.
CE-MRA is the MRA technique of choice to evaluate carotid artery disease ( Fig. 44.1 ). Typically, 3D CE-MRA data are acquired in the coronal plane. Bolus timing is essential, because venous return in the veins of the neck is quite fast. Spatial resolution of 0.71 mm 3 at 1.5 T and 0.54 mm 3 at 3 T are recommended. Generally, single dose (0.1 mmol/kg) of a Gd-based contrast agent is sufficient to achieve excellent image quality and resolution at 1.5 T. Several studies showed that dose reduction to half dose (0.05 mmol/kg) is feasible at 3 T without compromising SNR and spatial resolution.
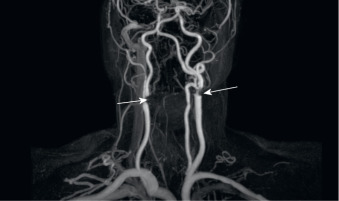
In several studies, time of flight MRA showed good sensitivity and specificity in detecting stenosis of the carotid bifurcation. However, CE-MRA offers high-resolution imaging of larger field of view in shorter acquisition times and therefore, has largely replaced time of flight MRA in clinical practice.
Development of new non–CE-MRA sequences show promising results and could have the potential to become an alternative imaging approach in evaluating carotid artery disease. Zhoa et al. showed that 3D black-blood CMR imaging provided high agreement for stenosis measurements as compared with DSA. Three-dimensional black-blood CMR imaging depicted longer lesion length than DSA, because of its ability to define vessel wall morphology and distribution of plaque. When compared with CE-MRA, 3D black-blood MRA shows accurate measurement of carotid stenosis and sensitivity and specificity comparable with CTA.
Other vascular disease of the carotid artery can also be diagnosed by CE-MRA. Fibromuscular dysplasia is a nonatherosclerotic, noninflammatory arteriopathy with segmental involvement of small-sized and medium-sized arteries. The etiology is unknown and the extracranial carotid, vertebral, and renal arteries are most frequently affected. The so-called string-of-beads sign of the involved arteries is a classic pattern seen in fibromuscular dysplasia, but also other imaging findings are described as aneurysm, fusiform arterial ectasia, and vascular loops. Fibromuscular dysplasia can accurately be diagnosed using CE-MRA; however, a negative result on CE-MRA does not exclude fibromuscular dysplasia. Therefore DSA is still recommended in patients with high clinical suspicion of fibromuscular dysplasia.
Carotid artery dissection is one of the most common causes of stroke in young patients. Dissection may occur after trauma or may arise spontaneously. In patients suspected for carotid artery dissection, CE-MRA is the imaging modality of choice. Mural hematoma can be visualized by performing additional axial T1 weighted images with fat suppression. Although DSA is the reference standard for evaluation of the lumen of the carotid artery, it does not provide details of the arterial wall. In suspected cases it is important to reformat acquired high-resolution isovolumetric voxels in axial thin slices to eventually depict an intimal flap.
Aortic Magnetic Resonance Angiography
Diseases of the thoracic and abdominal aorta can be examined by several imaging modalities. Over the last decade, noninvasive imaging techniques have improved significantly and therefore the need for DSA as an imaging tool in clinical practice has decreased. With noninvasive techniques, the risks of arterial catheterization and the use of iodinated contrast medium can be avoided.
A wide spectrum of vascular diseases affecting the thoracic and abdominal aorta can be evaluated by angiographic imaging, including aortic aneurysm, suspected aortic dissection, penetrating atherosclerotic ulcer, atherosclerotic arterial occlusive disease, genetic diseases (e.g., Marfan syndrome), and congenital abnormalities such as coarctation of the aorta. For imaging the thoracic aortic lumen, current established noninvasive techniques such as transthoracic echocardiography (TTE), transesophageal echocardiography (TEE), MRA, and CTA are able to provide additional information about the aortic wall and the relationship of vascular disease to surrounding tissue. TTE is the most common first-line imaging modality, but MRA and CTA are widely used. The abdominal aorta is most typically imaged by ultrasound, MRA, and CTA, whereas TTE is the most frequently used imaging modality for evaluating the aortic root and proximal ascending aorta.
In a standardized TTE examination the thoracic aorta is routinely assessed. Despite the fact that TTE is limited for assessing the complete thoracic aorta, it still permits adequate assessment of several aortic segments, especially when monitoring aortic root diameters. Moreover, TTE can be used as a screening tool for proximal ascending aorta dilatation, but is not sensitive for detection of aortic dissection, particularly beyond the proximal ascending aorta; however TTE is quite useful to assess for associated complications of type A dissections (e.g., aortic valve disruption/regurgitation, hemopericardium).
Because of the proximity of the esophagus to the thoracic aorta, TEE is often used in thoracic aorta assessment and overcomes several of TTE limitations. TEE reveals high resolution images and, unlike TTE, allows gradual assessment of the thoracic aorta from the aortic root to the descending aorta. However, if TTE and/or TEE reveal inconclusive information or abnormalities are present, another imaging modality is required for confirmation/further evaluation.
CTA is an important imaging technique in vascular diseases of the thoracic and abdominal aorta. Especially in acute aortic syndrome (i.e., aorta dissection, intramural hematoma, penetrating atherosclerotic ulcer, and contained aortic rupture) CTA plays a key role and is the preferred primary imaging technique because of its speed, wide availability, and high spatial resolution. As a result, CTA has essentially replaced invasive DSA. Diagnostic accuracy of CTA for the detection of dissection and traumatic injury is excellent. Sensitivity and specificity for the detection of dissection are 100%. Steenburg et al. reported a sensitivity of 96%, a specificity of 99.8%, and accuracy of 99.8% for multislice CTA as compared with DSA in patients with acute thoracic aortic injury. False positives have been described with CTA if ECG gating is not performed.
The role of MRA in the evaluation of acute aortic syndrome is limited. Monitoring acute, unstable patients in the CMR scanner is more complicated and acquisition times are longer. However, MRA has several advantages over CTA, such as the previously mentioned radiation-free nature of the imaging modality in which the need for iodinated contrast medium is avoided. Therefore MRA is highly suitable for evaluation of aortic disease in young patients and for follow-up studies.
CE-MRA has emerged as the preferred MRA technique for evaluation of thoracic and abdominal vascular pathology independent of blood flow. High-resolution 3D image acquisition permits multiplanar and MIP reconstructions in any desired plane for detailed image evaluation ( Fig. 44.2 ). Three-dimensional CE-MRA acquisition does not require ECG triggering and can be performed during a single breath-hold of 15 to 20 seconds.
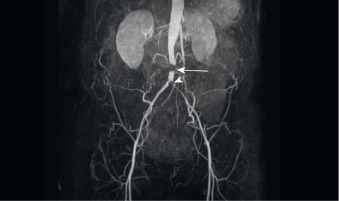
For optimizing SNR, surface phased array coils are used for imaging the thoracic and abdominal aorta, although sufficient image quality can often be achieved with the body coil. Positioning of the surface phased array coil in thoracic aorta imaging is important because the volume stack should include the proximal brachiocephalic arteries. When imaging the abdominal aorta, positioning of the 3D volume is essential so that the acquired volume contains the proximal course of major arterial braches, such as the cephalic trunk, superior mesenteric artery, renal arteries and iliac arteries.
For both the thoracic and abdominal aortic CE-MRA, patients are routinely imaged in supine position.
The imaging protocol of the thoracic aorta consists of a survey or localizer sequence, bSSFP cine imaging, contrast bolus timing sequence (test bolus or fluoroscopic real-time imaging), 3D CE-MRA sequence precontrast and postcontrast and, depending on vascular disease, T1-weighted postcontrast sequence to rule out, for example, aortitis. Single-shot fast spin echo or bSSFP acquired during breath-holding can serve as a localizer sequence in three directions. For optimal planning and positioning of the 3D CE-MRA imaging volume, it is essential that the survey scan is performed in the same breath-holding position as used for the 3D CE-MRA acquisition. Preferably, this breath-holding is end-expiration because it is more reproducible when compared with end-inspiration. bSSFP images are acquired with ECG triggering and during breath-holding. Preferably, two directions (axial and parasagittal planes) are acquired. bSSFP images provide additional information about the vessel wall and surrounding tissue.
The precontrast 3D scan is acquired to verify proper positioning of the 3D volume and to exclude possible aliasing artifacts. For 3D CE-MRA, 0.1 to 0.2 mmol/kg body weight Gd contrast is administered. The 3D dataset can be obtained in coronal, sagittal, or parasagittal plane. Covering the thoracic aorta in a coronal plane requires a larger volume and consequently, longer breath-holding maintaining high spatial resolution. Therefore in clinical practice, sagittal or parasagittal plane is favored. Typically, the field of view ranges from 30 to 40 cm.
Usually, the abdominal aorta is imaged in the coronal plane and a field of view of 35 and 40 cm. For both thoracic and abdominal CE-MRA, spatial resolution of at least 1 to 1.5 mm is recommended.
In CE-MRA of the thoracic and abdominal aorta, optimally two postcontrast datasets are obtained. Large aneurysms or false lumen in aortic dissection may have slow flow and therefore require a longer time interval between contrast injection and 3D image acquisition to become fully enhanced. As a consequence, they may be only fully established during the second 3D volume acquisition. Moreover, distinction between slow flow and therefore potential nonfilling with contrast of the lumen can be better distinguished from thrombus.
Additionally, ECG-triggered T1-weighted images with fat suppression can be acquired to analyze the aortic wall. Phase-contrast sequences can be performed to quantify flow.
Non–CE-MRA of the thoracic aorta can also provide additional information about the aortic wall and surrounding tissue, and therefore this acquisition is an important sequence within the standard MRA imaging protocol. Non–CE-MRA by bSSFP may serve as a useful alternative for evaluation and follow-up of aortic disease when compared with CE-MRA in patients with contraindications to Gd use or with poor intravenous access. bSSFP showed high diagnostic accuracy for aortic dimensions and vascular pathology, and similar reader confidence, when compared with CE-MRA. For the detection of aortic disease, free breathing 3D SSFP MRA with navigator gating showed a 100% diagnostic accuracy with CE-MRA serving as reference standard. Furthermore, bSSFP showed significantly higher image quality of the aortic root, whereas non–ECG-gated CE-MRA images may suffer motion artifacts in this region hindering diagnostic image evaluation.
For CE-MRA, postprocessing of the acquired 3D dataset is essential. On MPR and MIP reconstructions aortic vascular pathology can be assessed in relation to the origin of branching vessels. For serial follow-up examinations in patients with aortic dilatation, consistency and accuracy of aortic diameter measurements is critical, because increase in diameter may have important impact on risk stratification and on planning of surgical intervention. The maximal diameter should be measured perpendicular to the axis of flow, double oblique or so-called centerline reconstruction. Double oblique measurements of aortic diameter provided better agreement with the reference standard of planimetry than axial measurements and, therefore, may have potentially important impact on surgical decision-making.
An aneurysm of the thoracic aorta is often an incidental finding during TTE for another indication or on chest x-ray in asymptomatic patients. Aortic aneurysm may lead to aortic rupture or aortic dissection. These acute events may be rapidly fatal in a large proportion of patients ; therefore serial follow-up examinations in this patient population are vital. CE-MRA is a robust, accurate, and reproducible imaging modality for evaluation of the entire aorta and is therefore favorable for long-term monitoring examinations because it does not employ ionizing radiation or iodinated contrast ( Fig. 44.3 ).
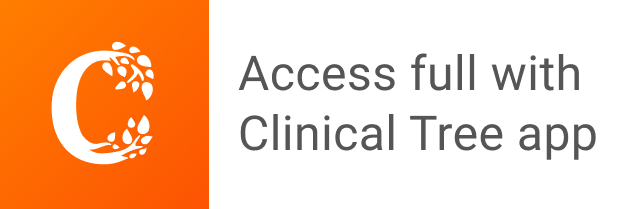