Molecular imaging examinations using positron emission tomography (PET) and single-photon emission computed tomography (SPECT) are frequently used in the brain because they can complement the anatomical information from magnetic resonance (MR) imaging and computed tomography (CT). By examining cellular function, disease is often detected at an earlier stage, or the extent of disease may be more accurately demonstrated with nuclear medicine techniques. Hybrid cameras combining PET and SPECT with CT or MR (PET/CT, SPECT/CT, and PET/MR) allow optimal image acquisition so that structural and physiological data can be accurately correlated. Some indications for nuclear medicine imaging in the central nervous system (CNS) include the following: characterization of dementia, diagnosis of parkinsonian syndromes, presurgical seizure focus localization, confirmation of tumor recurrence, brain death identification, stroke risk evaluation in vascular disorders (e.g., following carotid occlusion or in Moya Moya), and assessment of cerebrospinal fluid (CSF) flow or ventricular shunt function. The most common indications are listed in Box 14.1 .
Epileptic seizure focus identification
Dementia diagnosis
Parkinson’s disease/parkinsonian syndrome differentiation from essential tremor
Recurrent glioma
Differentiate tumor from opportunistic infection in ring-enhancing lesions
Vascular reserve assessment in patients at high stroke risk
Acute stroke: select candidates for thrombolytic therapy
Brain death diagnosis
Ventricular shunt function
Normal-pressure hydrocephalus
Cerebrospinal fluid leak
Investigational
Psychiatric diseases
Head trauma
Neuroinflammation
Tau and other proteinopathies in various types of dementia
The blood–brain barrier (BBB) makes imaging the CNS more complicated. When diseases such as glioblastoma disrupt the BBB, traditional agents, such as thalium-201 (Tl-201) and technetium-99m (Tc-99m) sestamibi, can be used. The intact BBB, however, prevents most radiopharmaceuticals from entering the brain ( Fig. 14.1 ). There are two lipophilic SPECT agents currently available clinically, Tc-99m hexamethylpropyleneamine oxime (Tc-99m HMPAO) and Tc-99m ethyl cysteinate diethylester (Tc-99m ECD), that are able to traverse the barrier and are taken up in viable neurons. The most commonly used PET agent, F-18 fluorodeoxyglucose (F-18 FDG), is a glucose analog and is actively taken up by the brain using the same pathways as the glucose that the brain requires for fuel. Other radiotracers have been created that are able to exploit other uptake mechanisms, such as those for key amino acids. For example, imaging nigrostriatal function in Parkinson’s disease can be performed with F-18 F-DOPA PET or with the SPECT dopamine transporter (DAT) analog iodine-123 ioflupane (I-123 DaTscan).
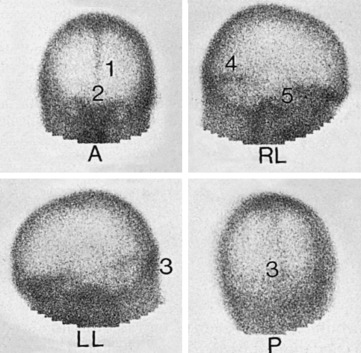
PET imaging has made significant contributions to our understanding of dementia. The impact of PET radiopharmaceuticals approved for the detection of abnormal beta-amyloid protein deposits (F-18 florbetapir, F-18 flutemetamol, F-18 florbetaben) is being investigated in multicenter trials looking at Alzheimer’s and mild cognitive impairment (MCI). Other proteins that accumulate in the brain in other dementia subtypes, such as the protein Tau, are also being explored with targeting radiotracers. Additional imaging biomarkers are being sought for neuroinflammation and other processes that contribute to neurodegenerative diseases.
This chapter reviews commonly performed scintigraphic brain-imaging procedures. Radiopharmaceuticals able to cross the intact BBB for clinical use in PET and SPECT are listed in Table 14.1 . Several experimental PET agents are listed in Table 14.2 .
Agent | Localizing Mechanism | Use |
---|---|---|
F-18 fluorodeoxyglucose (F-18 FDG[ED2]) | Glucose metabolism: Cellular activity and viability |
|
Technetium-99m (Tc-99m) hexamethylpropyleneamine (Tc-99m HMPAO, Ceretec) Tc-99m ethyl cysteinate dimer (Tc-99m ECD, Neurolite) | Perfusion and metabolism mirror cellular activity and viability |
|
I-123 Ioflupane (DaTscan) | Presynaptic dopamine transporter (DAT) binding | Diagnose parkinsonian syndromes/Parkinson’s disease |
F-18 florbetapir (F-18 AV-45) F-18 florbetaben (F-18 AV-1) F-18 flutemetamol (F-18-3′-F-PIB) | Beta-amyloid (Aβ) deposition | Excluding or identifying potential Alzheimer’s disease |
Thallium-201 (Tl-201) Tc-99m sestamibi (MIBI) | Nonspecific uptake relates perfusion and activity
| Differentiating recurrent brain tumor from MRI-enhancing posttreatment scar |
Agent | Imaging Target |
---|---|
C-11 PIB | Beta-amyloid (A[ED3]β) deposition |
F-18 FDDNP | Aβ, tau, other proteins (e.g., Huntingtin) |
F-18 flortaucipir (F-18 AV-1451) | Tau deposition |
F-18 MISO | Tumor hypoxia |
Cu-64 ATSM | |
F-18 fluorothymidine (F-18 FLT) | DNA synthesis/tumor diagnosis |
F-18 fluoroethyl L-tyrosine thymidine ( 18 F-FET) | Amino acid metabolism/peptide synthesis/tumor diagnosis |
C-11 methionine | |
F-18 fluoro- l -dopa | Peptide synthesis (tumor) and Neurotransmitter (clinical use at limited sites) |
C-11 dihydrotetrabenazine (C-11 DTBZ) | Vesicular monoamine transporter 2 |
F-18 fluoropropyldihydrotetrabenazine (F-18 DTBZ) | |
C-11 raclopride F-18 fallypride | D2/D3 dopamine receptor activity |
C-11 carfentanil | Mu opiate receptor activity |
F-18 FDPN | Opiate receptor (nonspecific) activity |
C-11 flunitrazepam | Benzodiazepine receptor activity |
C-11 scopolamine | Muscarinic cholinergic receptor activity |
C-11 ephedrine | Adrenergic terminals |
O-15 H 2 O | Blood flow |
O-15 O 2 | Oxygen metabolism and flow |
O-15 or C-11 carboxyhemoglobin | Blood volume |
Knowledge of brain anatomy is critical in understanding patterns of disease and image interpretation. The brain consists of two hemispheres, further segmented into lobes, above the tentorium and the cerebellum below in the posterior fossa. The lobes of the brain are illustrated in Fig. 14.2 . Within these lobes, key functional centers, or regions, have been identified that are important when trying to assimilate clinical changes with anatomical and functional images ( Fig. 14.3 ). Studies such as dynamic radionuclide brain flow and brain death examination allow visualization of the vascular supply of the brain to a limited degree, so understanding the arterial and venous anatomy is important ( Figs. 14.4 and 14.5 ). Even more important for image interpretation is familiarity with the cerebral regions these vessels supply ( Fig. 14.6 ).
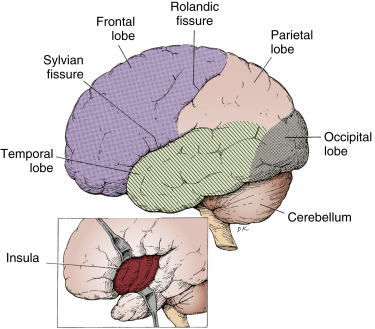
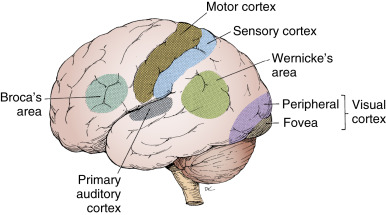
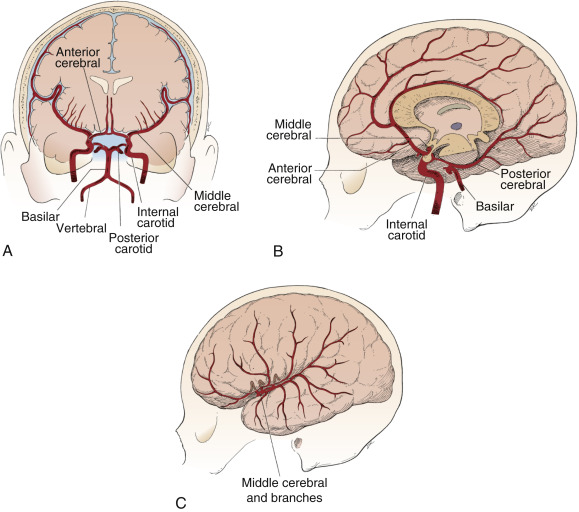
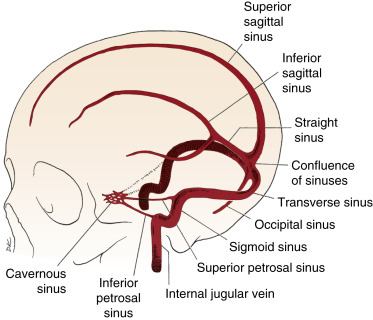
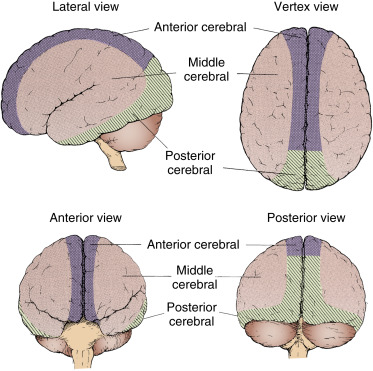
Radiopharmaceuticals
The brain is an obligate glucose user, and it is possible to determine the level of activity in different areas of the brain when imaging with the glucose analog F-18 FDG. The level of regional cerebral glucose metabolism (rCGM) usually closely correlates with regional cerebral blood flow (rCBF), and both parameters reflect activity in the neuron.
F-18 FDG is able to cross the BBB using glucose transporter systems. After entering the neuron, rapid phosphorylation by hexokinase-1 occurs. F-18 FDG cannot proceed further along the glucose metabolism pathway, and once phosphorylated, it cannot cross back through the cell membrane. Approximately 4% of the administered dose is localized to the brain. By 35 minutes after injection, 95% of peak uptake is achieved. Urinary excretion is rapid, with 10% to 40% of the dose cleared in 2 hours. F-18 FDG can be shipped from regional cyclotron production facilities due to its 110-minute half-life. As with all PET emitters, its decay results in two high-energy 511-keV gamma photons traveling at 180 degrees, which are best detected by a dedicated PET/CT or PET/MR camera.
The two SPECT agents currently used to assess regional cerebral blood flow are Tc-99m HMPAO and Tc-99m ECD. Favorable characteristics of these two neutral, lipophilic agents include high first-pass extraction across the BBB, distribution corresponding to rCBF, and desirable 140-keV gamma photons. However, both slightly underestimate true rCBF, especially at high-flow states. The Tc-99m perfusion agents are relatively fixed once inside in the neuron. Therefore images can be delayed and still show what the perfusion pattern looked like at the time of injection. For example, if the agent is injected during an epileptic seizure, images can be performed after the seizures are brought under control (within a few hours).
Tc-99m HMPAO (Tc-99m exametazime [Ceretec]) was first introduced in the mid-1980s. It was originally available as a kit requiring use within 30 minutes of radiolabeling; however, stabilizers have since been added, allowing a 4-hour shelf life after addition of the radiolabel. Doses from the radiopharmacy should be labeled with fresh generator eluate (<2 hours old) just before delivery. Tc-99m HMPAO has an approximately 80% first-pass extraction; 3.5% to 7% of the injected dose localizes in the brain within 1 minute of injection. Once across the BBB, it enters the neuron and becomes a polar hydrophilic molecule trapped inside the cell. However, some of the radiopharmaceutical may be present in different isomeric forms that are not trapped. Although up to 15% of the dose washes out in the first 2 minutes, little loss occurs over the next 24 hours. SPECT images can be acquired from 20 minutes to 2 hours after injection. Excretion is largely renal (40%) and gastrointestinal (15%).
Tc-99m ECD (Tc-99m bicisate, Neurolite) is a neutral lipophilic agent that passively diffuses across the BBB like Tc-99m HMPAO. Once prepared, the Tc-99m ECD dose is stable for 6 to 8 hours. It has a first-pass extraction of 60% to 70%, with peak brain activity reaching 5% to 6% of the injected dose. The blood clearance is more rapid than Tc-99m HMPAO, resulting in better brain-to-background ratios. At 1 hour, less than 5% of the dose remains in the blood, compared with more than 12% of a Tc-99m HMPAO dose.
Once inside the cell, Tc-99m ECD undergoes enzymatic deesterification, forming polar metabolites unable to cross the cell membrane. However, slow (roughly 6% per hour) washout of some labeled metabolites occurs, with almost 25% of the brain activity cleared by 4 hours. Although images may be superior to those with Tc-99m HMPAO at 15 to 30 minutes after injection, they may be suboptimal if imaging is delayed.
PET Image Acquisition
Patient preparation for F-18 FDG brain imaging is similar to that for oncology applications. Patients should fast for 4 to 6 hours before injection, have serum glucose less than 200 mg/dL, have had insulin withheld for 2 hours (short-acting insulin) to 8 to 12 hours (long-acting insulin), and avoid strenuous exercise for a few days before the test. Exercise and insulin cause radiotracer to be shunted into muscle, decreasing activity in the brain.
F-18 FDG should be injected in a quiet, dimly lit room with the patient remaining still and undisturbed during the uptake period (at least 30-35 minutes). CT images are limited, particularly in the posterior fossa, because low-dose protocols are used and the fixed camera gantry angle contributes to noise and streak artifact, respectively. The posterior fossa also poses a problem when PET/MR is used if calculated attenuation correction is not performed properly. Without the CT for attenuation correction, mathematical or atlas-based attenuation-correction methods are used that could result in substantial artifacts on the PET. Although time-of-flight technology is commonly used on the latest generation of scanners, this technique is less helpful than in the body, where scatter and attenuation are generally greater. A sample protocol is listed in Box 14.2 .
Patient Preparation
- •
Patient should fast 4 to 6 hours, avoid carbohydrates, and maintain normal blood glucose.
- •
Encourage oral hydration.
- •
If patient takes insulin, delay injection until effects have worn off (e.g., 2 hours after short-acting insulin, 8-12 hours for long acting).
- •
Check blood glucose. If less than 180 to 200 mg/dL, continue.
- •
Elevated glucose: Consider rescheduling, administer insulin, recheck, and delay 2 hours.
- •
If patient experiences seizures, an interictal scan is recommended. EEG may be used to avoid erroneous interpretation in postictal or occult ictal periods.
- •
If conscious sedation is required, administer short-acting benzodiazepine >20 minutes after injection, as close to scan acquisition as possible.
Radiopharmaceutical
- •
F-18 FDG 8 mCi (296 MBq) intravenous (IV) (range 5-12 mCi [185-444 MBq])
- •
Inject in a quiet, dimly lit room, with the patient’s eyes open.
- •
Wait 35 to 60 minutes (adhere to this same delay on any subsequent scan).
Image Acquisition
- •
Low-dose CT scan for attenuation correction/anatomical localization (contrast optional)
- •
Acquisition: 7 to 12 minutes per bed position for one bed position
- •
Serial dynamic scans can be acquired if significant motion artifact is anticipated (e.g., five 2-minute frames).
Processing
- •
Orient brain along the anterior commissure–posterior commissure plane.
- •
Iterative (or analytic) reconstruction, automated software
- •
Pixel size < 2 mm
CT, Computed tomography; EEG, electroencephalogram; FDG, fluorodeoxyglucose; PET, positron emission tomography.
For perfusion brain SPECT exams, no particular preparation is needed. As with PET, patients are injected in a quiet, dimly lit room. For the best-quality image, a delay of 30 to 60 minutes for Tc-99m ECD and 30 to 90 minutes for Tc-99m HMPAO should be used to improve the signal-to-noise ratio. A gamma camera with multiple heads creates images superior to single-headed systems. Patient positioning is just as important as the equipment used. The heads of the camera must come as close to the patient as possible, or resolution is reduced. A head-holder attachment extending from the end of the table allows the camera heads to come in closer than the width of a table or the patient’s shoulders. In heavy patients and those whose shoulders obstruct the view, the posterior fossa may not be seen. The SPECT images can be processed with either iterative reconstruction or filtered back-projection. A filter is applied to smooth the image. In general, filters can be sampled and modified for each patient in the postprocessing stage to achieve an optimal image. A protocol for Tc-99m SPECT imaging is given in Box 14.3 .
Patient Preparation
None; start intravenous (IV) line in advance.
Radiopharmaceutical
Tc-99m HMPAO (Ceretec) or Tc-99m ECD (Neurolite)
Adults: 20 to 30 mCi (740-1100 MBq) IV
Pediatric dose: 0.2 to 0.3 mCi/kg (7.4-11.1 MBq[AU3]); minimum dose 3 to 5 mCi (111-185 MBq) IV
Make sure dose falls within recommended 6-hour shelf-life parameter.
Inject in a quiet, dimly lit room, with the patient’s eyes open.
Positioning
Maintain the minimum distance possible between camera heads and patient. A head-holder extension beyond the table end allows closer positioning of the camera heads.
Camera Setup
Orbit: Circular
Collimator: High resolution, parallel hole
Acquisition: Angle 3 degrees/step, 40 stops/head, 40 seconds/stop (total time, 27 minutes)
Computer: Matrix size: 128 × 128, zoom: 1.5 to 2 (for pixel size ≤ 3.5 mm)
Processing: Filtered back-projection or iterative reconstruction
Filter: Hamming, 1.2 high-frequency cutoff, or other low-pass (e.g., Butterworth) filter
Attenuation correction: Can be used
Tc-99m HMPAO SPECT images generally reflect cortical rCBF because it is determined by the oxygen demands of the brain. In addition, the blood-flow distribution is usually similar to the metabolism seen with F-18 FDG. Areas with more synaptic activity require greater blood flow. Activational studies can therefore target areas of the brain showing increased flow when stimulated by a certain task. Although findings on both SPECT and PET scans will closely correlate with neuronal activity, many studies have shown that the superior PET images are significantly more sensitive. Given this superiority, PET has largely replaced SPECT for several applications.
There is a 2:1 to 4:1 differential in uptake in gray matter compared with white matter. Lesions in the white matter are often undetectable or cannot be differentiated from adjacent CSF spaces, so magnetic resonance imaging (MRI) or CT correlation is necessary for identifying white-matter changes and enlarged ventricles. Although anatomy seen on CT and MRI is much more detailed, many structures are clearly visualized with scintigraphy ( Fig. 14.7 , A , D ). Typically, activity is fairly evenly distributed between the lobes of the brain. However, this is dependent on the conditions at the time of injection. For example, bright lights will increase occipital lobe activity, falsely causing the frontal lobes to appear decreased.
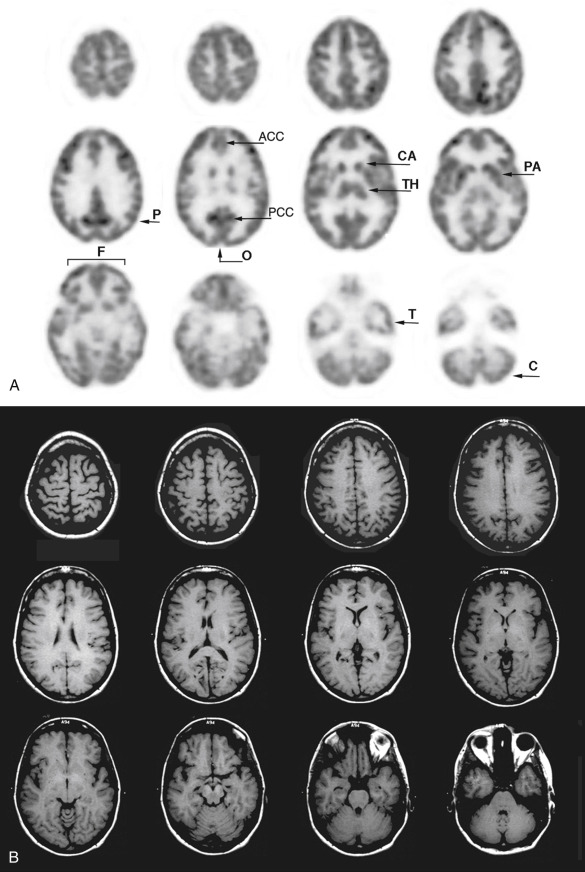
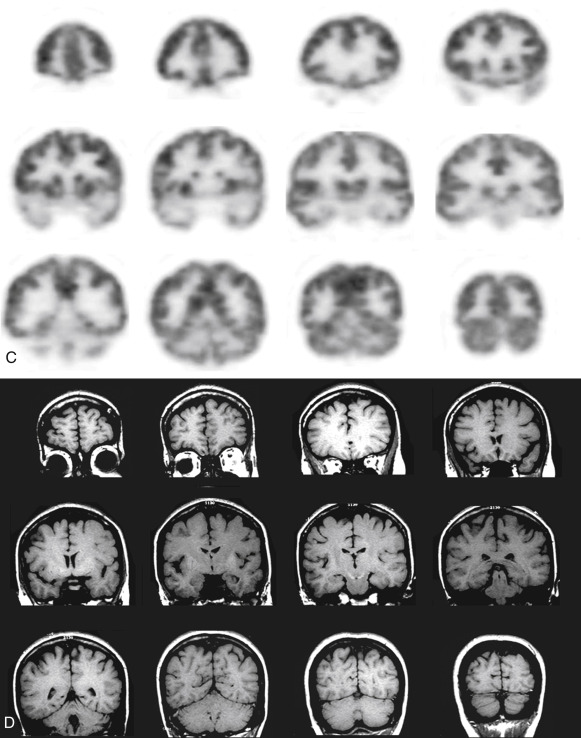
The distribution of Tc-99m HMPAO differs only slightly from that of Tc-99m ECD. Tc-99m HMPAO accumulates more in the frontal lobes, thalamus, and cerebellum, whereas Tc-99m ECD shows a higher affinity for the parietal and occipital lobes. Although the differences are not usually noticeable, it would be best to use the same agent for serial examinations, and most clinicians use the agent with which they are most familiar.
The normal distribution of SPECT and PET agents also changes with age. In infants, a relative decrease is seen in frontal lobe perfusion. This frontal activity increases over time, reaching an adult level by about 2 years of age. In adults, global activity decreases with age, and this decrease is more prominent in the frontal regions. Given these changes, using comparison age-matched normal databases and computer programs that quantitate rCBF may help improve interpretation accuracy.
Although dementia can be the result of many conditions ( Box 14.4 ), it is usually caused by a neurodegenerative disease ( Box 14.5 ). Neurodegenerative disorders, also referred to as proteinopathies, are a varied group of disorders caused by the buildup of neurotoxic, misfolded proteins over time. These disorders can be grouped not only on the basis of the symptoms ( Box 14.6 ) that they cause but also by the key proteins underlying each disorder as well ( Fig. 14.8 , A , B ).
Degenerative dementias/neurodegenerative proteinopathies
Vascular dementia: stroke or multiinfarct dementia
Vitamin B 12 deficiency
Medication (drug abuse, overdose, or from side effects)
Alcohol abuse
Depression
Thyroid disorder
Infection (e.g., HIV/AIDS, Lyme disease)
Subdural hematoma
Normal-pressure hydrocephalus
Brain tumor
Multiple sclerosis
Renal failure, heart disease, chronic obstructive pulmonary disease, dehydration
- •
Alzheimer’s disease (AD)
- •
Classic, late-onset “amnestic” AD
- •
AD variants: Posterior cortical atrophy, corticobasal syndrome/corticobasal degeneration, frontal variant AD, logopenic variant primary progressive aphasia
- •
- •
Frontotemporal dementia (FTD)/frontotemporal lobar degeneration (FTLD)
- •
Behavioral variant FTLD (e.g., Pick’s disease)
- •
Primary progressive aphasia (PPA): Semantic variant PPA, nonfluent variant PPA
- •
- •
Parkinsonian syndrome
- •
Parkinson’s disease (PD)
- •
Atypical parkinsonian syndromes: Progressive supranuclear palsy, multisystem atrophy, corticobasal syndrome
- •
- •
Dementia with Lewy bodies (DLB)
- •
Others:
- •
Chronic traumatic encephalopathy (CTE)
- •
Cerebral amyloid angiopathy (CAA)
- •
Down’s syndrome (trisomy 21)
- •
Huntington’s chorea
- •
Creutzfeldt–Jakob disease (prion diseases not always included)
- •
Dementia
Episodic memory loss, becoming lost in familiar places, difficulty performing multistep tasks
- •
Alzheimer’s disease (AD):
- •
Classic Alzheimer’s (amnestic AD): Memory loss—presents early in disease
- •
Typical late-onset form (≥65), sporadic (familial forms uncommon)
- •
Early-onset form (<60-65), may be familial, rapid progression compared with late-onset form
- •
AD variants: Amyloid-positive disorders, commonly early onset, with memory usually intact initially, instead presenting with other key features (see following points)
- •
Posterior cortical atrophy (visual variant), logopenic variant primary progressive aphasia (verbal variant), corticobasal syndrome (motor variant), behavioral or frontal variant AD
- •
- •
Dementia with Lewy bodies (DLB)
- •
Fluctuating decreased cognition, visual hallucinations, rapid eye movement sleep disorder
- •
Parkinsonism: Bradykinesia, rigidity, and/or rest tremor
- •
Supporting signs: Autonomic dysfunction, severe reactions to antipsychotics, falls/postural instability
- •
- •
Parkinson’s disease dementia (PDD): Frequent development in Parkinson’s disease (PD)
- •
PD, PDD, and DLB may represent a spectrum of disease and can overlap with AD.
- •
Personality and Behavior
Personality change, disinhibition, loss of judgment, apathy
- •
Behavioral variant frontotemporal lobar degeneration (bvFTLD)
- •
Frontal variant AD: Clinical presentation like FTLD, with underlying histopathology similar to AD (e.g., tau neurofibrillary tangles, amyloid)
Speech and Language
- •
FTLD primary progressive aphasia (PPA)
- •
Semantic variant primary progressive aphasia (svPPA)
- •
Inability to understand/remember meaning of words, understand sentences
- •
Nonfluent variant progressive aphasia (NFPA) or progressive nonfluent aphasia (PNFA)
- •
Ungrammatical and/or hesitant (nonfluent) speech, difficulty pronouncing/getting words out, speech may slur or voice may change
- •
- •
Alzheimer’s variant (verbal variant AD):
- •
Logopenic variant PPA (lvPPA) or logopenic variant progressive aphasia (LVPA)
- •
Deficits in naming objects and repetition, with sparing of semantic, motor, syntactic abilities
- •
Speech slows as patient searches for words, but word meanings are preserved, and speech is not physically effortful.
- •
Motor Abnormalities: Parkinsonism and Motor Neuron Pathology
- •
Parkinsonism: Resting tremor, rigidity, bradykinesia, falls, postural instability/balance issues
- •
PD
- •
Parkinsonism, olfactory loss, or cardiac sympathetic denervation
- •
Responds to dopaminergic medication
- •
Dementia frequent (PDD)
- •
Differentiating PD from atypical parkinsonian syndromes (APSs) may be clinically difficult.
- •
- •
DLB
- •
Dementia, visual hallucinations, parkinsonism
- •
- •
Multisystem atrophy (MSA)
- •
Atypical parkinsonian syndrome: Ataxia, long tract signs
- •
MSA-P: Parkinsonism symptoms dominate
- •
MSA-C: Cerebellar ataxia, autonomic nervous system dysfunction
- •
- •
Progressive supranuclear palsy (PSP)
- •
Most common of the APSs
- •
Oculomotor abnormalities and deficits in vertical gaze
- •
Rigidity, falls, gait disorder, bulbar symptoms
- •
- •
Corticobasal degeneration (see following points)
- •
Motor neuron/motor cortex
- •
Corticobasal syndrome (CBS): Term used for a constellation of symptoms, multiple potential etiologies
- •
Corticobasal degeneration (CBD): CBS with tauopathy at autopsy (causes 50% of CBS)
- •
Asymmetric, usually begins with one limb
- •
Motor cortex/premotor affected: Progressive rigidity and apraxia, alien limb phenomena
- •
Striatal (basal ganglia)/extrapyramidal dysfunction: Parkinsonism
- •
May also show: Myoclonus, dysphagia, visuospatial disorientation, acalculia, dementia
- •
Symptoms may overlap other neurodegenerative diseases with motor deficits (PSP, PD, and MSA)
- •
- •
Others:
- •
Amyotrophic lateral sclerosis (ALS): upper and lower motor neurons
- •
Huntington’s chorea
- •
Visual Abnormalities
- •
Visual field deficits
- •
Posterior cortical atrophy (PCA): Visual variant AD
- •
Presenting abnormalities from asymmetric, lateral occipital involvement; memory initially relatively preserved
- •
Asymmetric atrophy in the occipital lobe may be seen on magnetic resonance imaging
- •
- •
Visual hallucinations
- •
DLB
- •
AD: Later finding in up to 20%
- •
Sometimes seen in posterior cortical atrophy (PCA)
- •
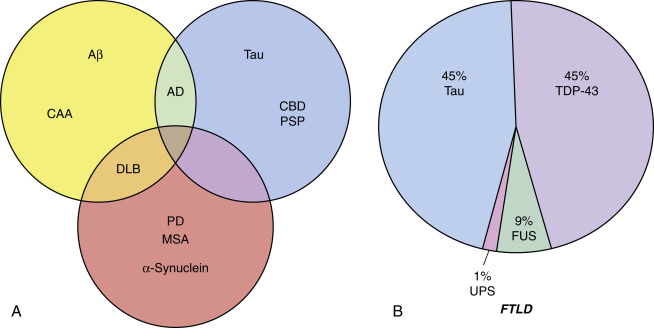
Abnormal Proteins in Alzheimer’s Disease
Alzheimer’s disease (AD) is associated with two abnormal proteins that make up the extracellular “senile” plaques and intracellular neural fibrillary tangles (NFT s ) found histopathologically. NFTs are composed of a protein called tau, whereas the plaques are largely made up of abnormally folded amyloid protein, beta-amyloid (also referred to as amyloid-β or Aβ). The abnormal configuration of Aβ causes fragments to autoaggregate, forming pleated sheets and insoluble fibrils.
The amyloid cascade hypothesis proposes that Aβ deposition is the central event that incites the neuroinflammation, NFT formation, vascular damage, and synaptic loss that ultimately lead to neuronal death in AD. This theory is supported by studies showing that the Aβ fibrils are neurotoxic. Additionally, the genetic defects found in familial forms of AD cause an increase in abnormal Aβ by altering amounts of the amyloid precursor protein (APP) or by changing the way APP is cleaved during posttranslational processing. The abnormal Aβ (i.e., Aβ42) fragments have a different configuration that leads to the auto-aggregation and formation of insoluble fibrils and pleated sheets found in Alzheimer’s.
In the search for a cure for AD, attention has focused on the extracellular fibrillar plaques in accordance with the amyloid cascade hypothesis. However, clinical trials with new therapies that correct amyloid buildup have so far failed to correct cognitive decline or alter the course of disease. Several other facts are difficult to explain if the amyloid cascade hypothesis is entirely correct. First, significant Aβ deposition is seen in 15% to 30% of cognitively normal elderly patients. Also, amyloid volume and distribution fail to reflect the stage or severity of symptoms. For example, anteromedial temporal lobe dysfunction develops very early in the course of AD, but plaques are not detected there until later (first occurring in the basal temporal lobe, anterior cingulate, and parietal operculum). Also, Aβ plaque appears decades before the disease is clinically manifest and plateaus many years before the symptoms peak.
Despite the conflicting information as to the importance of amyloid, detecting its presence with PET or measuring changes in CSF amyloid concentration can help with the diagnosis of dementia, particularly in differentiating AD from frontotemporal lobar degeneration (FTLD). Other contributing factors that could play a role in dementia include inflammation from overactive microglial cells, decreased phagocytosis of toxic debris, and toxicity related to the combination of abnormal proteins present. In AD, the relationship between abnormal Aβ and tau proteins is increasingly attracting interest.
The protein tau is normally involved in the regulation of intracellular transport by the cellular microtubules and is encoded for by the MAPT gene located on chromosome 17. NFTs are largely composed of an insoluble, abnormally phosphorylated (hyperphosphorylated) form of the microtubule-associated protein tau (MAPT or tau).
In AD, tau accumulates in a pattern that mirrors the progression of functional changes. Filaments are first found in the anteromesial temporal lobe and hippocampus, then spread to involve the lateral temporal and temporoparietal cortex. The posterior cingulate cortex (PCC) and superior posterior parietal lobe are next involved. Unlike amyloid, the amount of tau present appears to correlate with the severity of disease, and evidence increasingly points to tau as a critical factor in the development of the AD.
Different forms of tau accumulate have been found to be characteristic in several other neurodegenerative disorders. These disorders are cumulatively referred to as “tauopathies” and include the behavioral variant frontotemporal lobar dementia (bvFTLD), corticobasal degeneration (CBD), and progressive supranuclear palsy (PSP). In the past, FTLD was referred to as Pick’s disease, but that term is now used to refer to the subset of frontotemporal dementia (FTD) cases where Pick bodies, intracellular inclusions containing tau, are seen in tissue specimens.
Lewy bodies are another type of intracellular inclusion, composed largely of abnormal α-synuclein protein. Lewy bodies were originally described in Parkinson’s disease (PD), but they are also found in dementia with Lewy bodies (DLB) and Parkinson’s disease dementia (PDD). It is likely that DLB and PDD are related on a spectrum of disease. Neuronal involvement in each disease progresses in a predictable pattern marked by the spread of the abnormal protein. For example, Lewy bodies in DLB are found in the brainstem and substantia nigra before moving rostrally to dopaminergic neurons in the striatum. This is followed by the cingulate region, insula, and finally widely through the cortex. Lewy bodies are not specific to diseases of striatal or motor neurons and are found in other conditions, including 50% of patients with AD. In fact, the overlap between AD and DLB is significant in other ways, with patients with DLB frequently showing AD cellular pathology (i.e., amyloid), and the F-18 FDG PET patterns of the two disorders are sometimes difficult to differentiate in clinical cases. Abnormal α – synuclein can be found in forms other than as Lewy bodies. For example, glial intracellular inclusions, primarily in oligodendrocytes, are the hallmark of the atypical parkinsonian syndrome, multisystem atrophy (MSA).
Roughly 50% of FTLD cases are tau negative. The abnormal proteins related to these cases have been characterized recently, with two proteins being involved in almost all cases: TAR-DNA binding protein of 43 kDa (TDP-43) and fused in sarcoma (FUS) protein. These two proteins also often occur in a subset of amyotrophic lateral sclerosis (ALS), and accumulations of FUS are found in some patients with essential tremor. The two proteins can also be seen as secondary proteins in other degenerative diseases when more than one protein is present.
Abnormal protein accumulation characterizes several other diseases. In Huntington’s chorea, an abnormal protein, Huntingtin, is found. Prion disease, such as in Creutzfeldt–Jakob disease, will also result in abnormally folded protein deposition. The dementia of Down’s syndrome (trisomy 21) is associated with AD pathology because of the presence of the amyloid precursor protein gene on chromosome 21. In addition, traumatic brain injury that leads to chronic traumatic encephalopathy (CTE) is strongly associated with NFT deposition. Understanding the different abnormal proteins found in the dementias may help uncover new diagnostic tests and therapies.
AD typically occurs after age 60 to 65 and is characterized primarily by progressive cognitive decline with episodic memory loss, difficulty navigating familiar places, and organizational problems (i.e., amnestic AD). AD is the most common cause of dementia, accounting for 60% to 80% of cases diagnosed in the United States and affecting 10% of the population over 65 years of age and nearly 50% in those over 85 years of age. In those under 65, dementia is rare (comprising roughly 5% of diagnosed cases), but when it occurs, early-onset dementia is most commonly caused by AD. Roughly half the cases are caused by AD, and most of the remainder are a result of FTLD/FTD. Early-onset AD is more frequently associated with familial inherited patterns of disease and demonstrates a more aggressive clinical course than the classic late-onset form.
More than 80% of AD cases are sporadic, but in those cases of inherited forms of disease, three important autosomal-dominant genetic defects have been found ( Table 14.3 ). In nonfamilial and late-onset disease, a strong correlation has been found with abnormalities in the genes for apolipoprotein (ApoE). Specifically, an increased incidence of AD is seen in those who carry the ApoE ε 4 allele, and the risk increases when more than one copy of the ApoE ε 4 is present. In both early- and late-onset disease, defects result in the buildup of abnormal forms of Aβ related to these genes. Other factors that have been linked to Alzheimer’s are diabetes mellitus, elevated serum glucose/hyperinsulinemia, hypertension, and head injury.
Chromosome | Gene | Notes |
---|---|---|
Early-Onset Dementia (<60-65 years of age) | ||
21 | Amyloid precursor protein (APP[ED4]) gene mutations | Improper APP cleavage: ↑Aβ42 fragment (↑Aβ42 to ↑Aβ420 ratio). |
Trisomy 21: Triplicate genes and ↑ APP expression related to early-onset AD in Down’s syndrome | ||
14 | Presenilin 1 (PSEN1) | Both part of the γ-secretase complex that cleaves APP |
1 | Presenilin 2 (PSEN2) | |
Majority of early-onset familial AD mapped to chromosome 14 | ||
Late-/Senile-Onset Dementia (>65 years of age) | ||
19 | Apolipoprotein E (ApoE): 3 isoforms (ε 2 , ε 2 , ε 2 ) | ApoE 4 is the major risk for late-onset AD |
Found in 65%-80% of sporadic and familial cases | ||
Multiple copies E 4 ↑↑ risk 2 copies by age 85, 12%; 4 copies, 91% by age 85 | ||
E3 neutral risk E2 protective ↓ risk 50% | ||
Triggering receptor on myeloid cells 2 (TREM2) | Less common; expression limited to microglia; critical for response to injury/Aβ | |
Others (very rare) |
Occasionally, AD will present clinically in an atypical fashion with symptoms other than memory loss dominating early in the course of disease. These disorders include aphasia in logopenic primary progressive aphasia (verbal variant AD), visual deficits in posterior cortical atrophy (visual variant), corticobasal degeneration (motor variant), and personality or behavioral changes (frontal lobe variant). Although clinical findings and corresponding F-18 FDG PET (or Tc-99m HMPAO/ECD SPECT) imaging findings vary widely in cases of atypical AD, the AD variants demonstrate the same underlying histopathological findings typical of amnestic AD (i.e., increased Aβ and NFT buildup and decreased CSF Aβ or tau).
Different phases of disease are now recognized in AD: preclinical, prodromal mild cognitive impairment, and dementia from Alzheimer’s ( Table 14.4 ). Preclinical amyloid deposition begins years before patients demonstrate symptoms. Such patients may be identified by genetic markers or abnormal imaging or CSF biomarker findings. In the early stages of disease progression, memory and cognitive issues are usually not serious enough to affect daily living activities. These patients are said to have MCI. MCI is present in 15% to 20% of the population over 65 years of age and can be caused by multiple etiologies. Patients may not develop dementia in 35% to 40% of cases. However, patients with MCI are at high risk for the development of AD, with 15% of patients with MCI converting to AD in 2 years and more than a third in 5 years (up to perhaps half of the cases). When MCI is caused by the early stages of AD, F-18 FDG PET (or sometimes SPECT) will often reveal abnormal patterns similar to AD. Additionally, amyloid PET scans will usually be positive in these cases. Thus, these scans can help predict which MCI cases will progress to actual AD. Once neurologic deficits have worsened to a level that affects daily living activities, the clinical diagnosis of dementia is made.
Clinical | Biomarkers | ||||
---|---|---|---|---|---|
Category | Stage | Cognitive Change | Aβ PET or CSF | Neuronal Injury (Tau, MR, FDG) | |
Normal | 0 | AD biomarkers normal | – | – | – |
Preclinical AD | 1 | Amyloidosis | – | + | – |
2 | Amyloidosis and neurodegeneration | – | + | + | |
3 | Plus early cognitive change | + (subtle—doesn’t meet MCI criteria) | + | + | |
SNAP | Neurodegeneration without amyloid | – | – | + | |
MCI | Symptomatic predementia—daily living activities not affected | + (mild) | + | + | |
Alzheimer’s Dementia | Symptomatic dementia | Affects daily living activities | + | + | + |
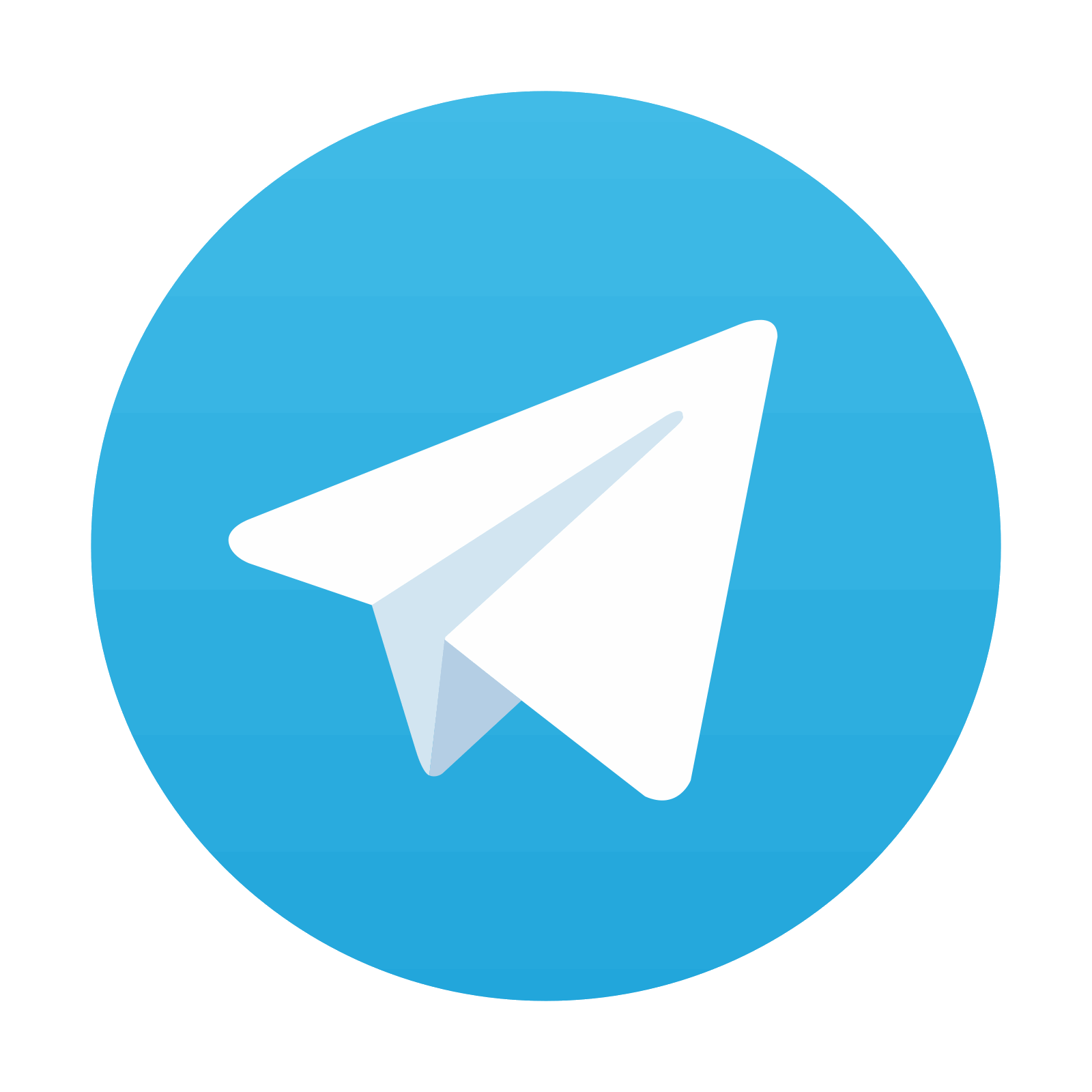
Stay updated, free articles. Join our Telegram channel
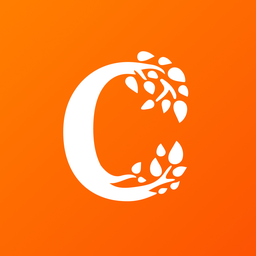
Full access? Get Clinical Tree
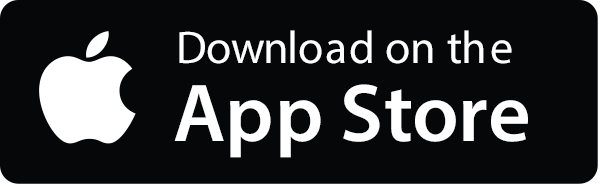
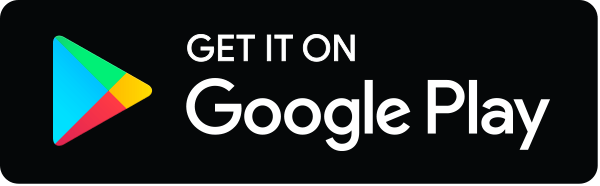
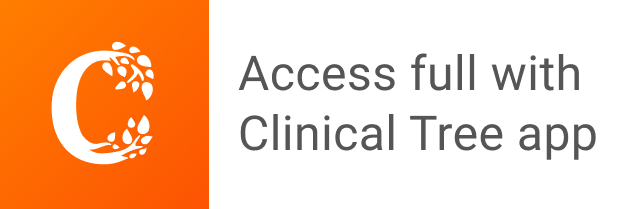