Fig. 3.1
Basic modular design of particle therapy systems
The beam production system is the “engine” of the particle therapy system. It is responsible for the acceleration of particles to energies capable of reaching the depths required for radiation therapy. Commonly used beam production systems include cyclotrons and synchrotrons, whereas newer designs such as linear accelerators or dielectric wall accelerators have also been proposed, but are currently still not used in any particle therapy systems.
In this chapter, we first introduce the basic properties of accelerators and cyclotrons to the reader. It is important to understand the difference between the two main families of cyclotrons: the isochronous cyclotron and the synchrocyclotron. In the second section, we examine how cyclotrons started to be used for medical applications: because they were easily available and they still constitute the best compromise for particle therapy purposes. The third section is devoted to currently available cyclotrons for particle therapy. The last section discusses future trends.
3.1 Accelerator Basics
Accelerators use static or oscillating radio frequency (RF) electric fields to accelerate electrically charged particles like protons, electrons, etc. The kinetic energies obtained from electrostatic accelerators are too low for proton therapy applications. Oscillating RF electric fields overcome this limitation.
In a linear accelerator (linac), the radio frequency accelerating electrodes are placed along a straight line (Fig. 3.2).
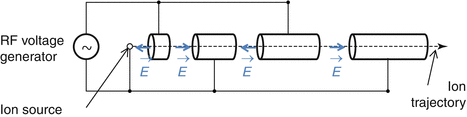
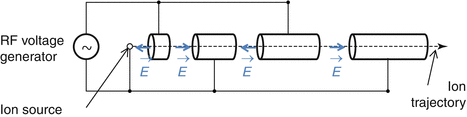
Fig. 3.2
Simple illustration of the linear accelerator (Wideroe structure)
For proton beams to be used for radiation therapy purposes, there is a need to accelerate protons up to 230 MeV. This will allow for the proton beam to reach a depth of 32 g/cm2. To achieve this energy, a relatively long linear accelerator with many accelerating RF cavities is needed. This will take a large footprint and uses significant electric wall plug power. In addition, the linear accelerator technology is considered as rather expensive compared to other solutions.
To reduce the size of the accelerator, one could imagine “winding” it into itself, using magnetic fields to bend the particle trajectories. In 1932, E.O. Lawrence [1] realized that a particle moving in a constant magnetic field moves on a circular trajectory at a frequency that is independent of its energy: the same particle, in the same magnetic field but with two different energies simply, has two circular trajectories with different radii but the same orbital frequency!
The revolution frequency, called the cyclotron frequency, is proportional to the charge q and the magnetic field, B, and inversely proportional to the particle mass, m:

(for a proton in a 1 T magnetic field, f ~ 15 MHz).

(3.1)
Based on this idea, the multiple cavities used in the linac can be merged into a single cavity. The required RF-frequencies are relatively low (as compared to a linac), and therefore the electric fields can be created continuously, and this new accelerator, named the cyclotron, provides a continuous wave (CW) beam.
The cyclotron accelerating structures (electrodes) are called “dees” because of their letter “D”-like shape in the early days. The dees act as a Faraday cage that screens the electric fields inside and confines them only to the accelerating gaps between the two dees. The ion is accelerated each time it crosses this gap. As a result of the acceleration, the curvature radius of the particle will increase, and its trajectory will be spiral shaped (Fig. 3.3). This is the key point to understanding the beauty of the isochronous cyclotron: it results in a compact accelerator using a single magnet and a single accelerating cavity operating at a constant frequency.
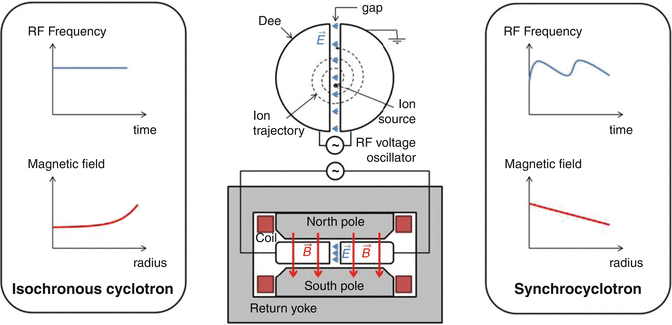
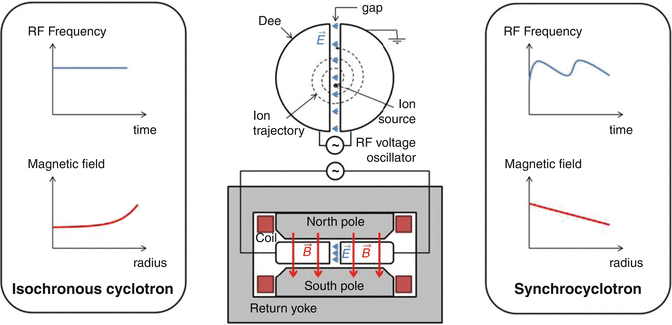
Fig. 3.3
Simple illustration of the isochronous cyclotron and synchrocyclotron
However, when the speed of the particle increases, the relativistic effects on the particle are no longer negligible (for protons: 1 % at 10 MeV, 11 % at 100 MeV, and 25 % at 230 MeV), and the mass increase of the particle decreases the value of its revolution frequency, and the particle goes out of phase (desynchronized) with respect to the RF system.
In the beginning of the cyclotron era, relativistic effects were not taken into account, and the problem of accelerated particles going out of synchronism had limited the final kinetic energies that could be achieved. Historically, the first solution proposed to obtain higher energies was to keep the rotationally symmetric magnetic field as before and to reduce the frequency of the RF system during the acceleration in order to compensate the mass increase. This idea was proposed in 1945 independently by Veksler [2] and by McMillan [3]. A new category of accelerator was born: the synchrocyclotron or the frequency modulated (FM) cyclotron (Fig. 3.3). The synchrocyclotron solution helped to overpass the previous kinetic energy limit of accelerated particles.
Besides the cyclotron and the synchrocyclotron, the synchrotron is the third type of circular accelerator used in particle therapy. This accelerator was invented simultaneously with the synchrocyclotron also by Veksler [2] and by McMillan [3]. In this case, the orbit trajectory is kept constant during acceleration, and the beam travels in a quasi-circular ring of bending magnets (Fig. 3.4). During the acceleration, the revolution frequency increases, and therefore a simultaneous increase of the magnetic field is needed as may be deduced from Eq. (3.1). Hence, both the bending magnetic field and the frequency of the RF system need to be pulsed.
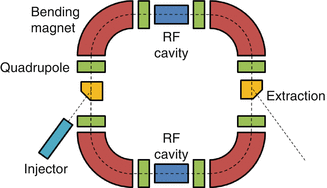
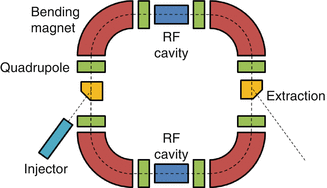
Fig. 3.4
Simple illustration of the synchrotron
Both cyclotrons and synchrocyclotrons can accelerate the beam starting from the (internal) ion source (very low kinetic energy) to the final kinetic energy in one single stage. For synchrotrons, this is not possible. A first pre-accelerator (also called an injector) is needed to speed up the particle to typically 1–7 MeV and then inject the beam into the synchrotron.
The typical synchrotron consists of a number of discrete dipole magnets and focusing quadrupoles, whereas cyclotrons or synchrocyclotrons usually have just one magnet. Therefore, the footprint of a synchrotron-based proton therapy facility is often larger than for a cyclotron facility. Nevertheless, Hitachi, a supplier of synchrotron-based proton therapy systems, is developing a very compact synchrotron [4] of 5.1 m diameter compared to 7.8 m in the previous design.
Besides the required condition of synchronism (or longitudinal stability) between the particle and the RF fields, the requirement of vertical focusing and stability is also crucial for the three types of circular accelerators that were discussed. Different methods of vertical focusing are possible: weak focusing, sector focusing, and strong focusing.
The method of weak focusing is used in a synchrocyclotron: it relies on the slow decrease of the rotationally symmetric magnetic field with increasing radius. In isochronous cyclotrons, the average magnetic field has to increase with radius to compensate for the relativistic mass increase. A rotationally symmetric field therefore would not provide any focusing.
Thomas [5] proposed a solution in 1938 (thus already 7 years before the invention of the synchrocyclotron and the synchrotron) consisting of a periodic magnetic structure of hills (also called poles) and valleys, as illustrated in Fig. 3.5. It deforms the orbit to a noncircular shape and creates vertical magnetic forces that push the particles back to the median plane at each entrance into and exit from a hill sector. The solution could not be applied immediately due to the increased complexity of the magnetic structure. That is the reason why synchrocyclotrons were widely used at the birth of proton therapy.
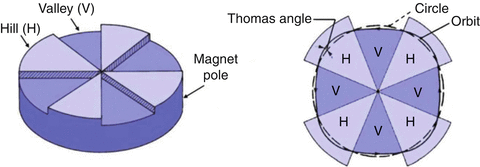
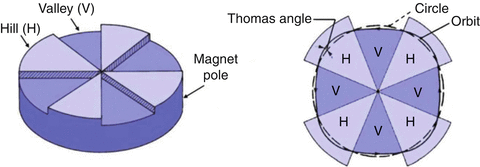
Fig. 3.5
Illustration of sector focusing in a cyclotron
Only about 20 years later, due to the availability of improved computational techniques, the Thomas concept allowed for the realization of the new family of isochronous cyclotrons. The first operation of such an azimuthally varying field (AVF) cyclotron was achieved in 1958 in Delft, Netherlands, by F.A. Heyn and Khoe Kong Tat [6].
For the development of the synchrotron, an important milestone was the discovery of the strong focusing (or alternating focusing) principle by Christofilos in 1950 [7] and by Courant, Livingston, and Snyder in 1952 [8]. Soon after that the same principle could be implemented in cyclotrons. This was done by replacing the radial (Thomas) sectors by spiral-shaped sectors. In the first step, this led to yet another type of circular accelerator called the FFAG (Fixed-Frequency Alternating Gradient) accelerator proposed by Kerst and Symon in 1954 [9]. One of the first spiral-sector isochronous cyclotrons was realized at Harwell [10].
An important difference between the three types of circular accelerators is the time structure of the beam. In all three cases, the micro-structure is determined by the RF-frequency. For an isochronous cyclotron, the RF-frequency is constant and therefore the macro-structure of the beam is CW (continuous wave). In the synchrocyclotron the beam is pulsed at a rate that is determined by the variable capacitor which modulates the RF-frequency as needed for the acceleration. The pulse rate can go up to a few kHz. For the synchrotron, the beam is also pulsed but at a much lower rate. This is due to eddy currents in the magnet iron and also the self-inductance of the magnets which limit their rise time. Repetition rates can go from 0.5 Hz for the slow cycling synchrotrons up to ~30 Hz for the fast cycling ones [11].
For pulsed operations, the beam intensity that can be obtained is generally lower than for continuous wave operations. This is due to the fact that only during a small fraction of the RF pulse can particles be trapped into stable orbits. This has been well explained in the papers of Bohm and Foldy [12, 13]. This intensity limitation was previously an important factor for passive scattering proton therapy systems as there was a need for the beam to pass through multiple scattering materials before reaching the tumor volume. However, this concern has decreased over time as most proton therapy systems are now treating with active scanning methods where the proton beam is directly deposited into the tumor volume via iso-energy slices. Such active scanning methods are also known as pencil beam scanning or spot scanning.
For pencil beam scanning, it is important to have fast and reliable control of the beam intensity as every spot to be delivered in the tumor volume has a different dose weight. This is relatively easy in the isochronous cyclotron where the current can be controlled directly at the ion source. For the synchrocyclotron, the beam intensity can be adjusted from pulse to pulse by adjusting the ion source or the RF voltage. As the pulse from a synchrocyclotron is of the order of kHz, this is also adequate for pencil beam scanning. For the synchrotron, the intensity adjustment needs to be done during the extraction from the ring within each pulse. As the pulse rate of the synchrotron is of the order of Hz, the rapid adjustment of its beam intensity is considered as more difficult than for cyclotron-based systems.
An advantage of the synchrotron is its variable energy feature. Recent developments show that the energy changes can be done from pulse to pulse within an RF cycle at 30 Hz [11], and the accelerator cycle can be synchronized with the respiratory cycle. Higher energies are easier to reach with the synchrotron making the possibility to go to 330 MeV for proton tomography. Cyclotrons and synchrocyclotrons are usually fixed energy machines and require an external energy degrader and an energy selection system (ESS) to reduce the proton energy from the nominal value (230 or 250 MeV) to lower energies with the minimum at 70 MeV convenient for shallow-seated tumors. The degrader (often made of carbon) produces secondary neutrons and gamma rays that must be shielded by the concrete walls. On the other hand, the energy degrader also provides a strong decoupling of the accelerator and beam transport line which makes control easier and more robust.
In Table 3.1 we summarize the discussion on the main similarities and differences between the three types of circular accelerators used for proton therapy.
Table 3.1
A brief overview of the main differences between the three commonly used circular accelerators for proton therapy
Isochronous cyclotron | Synchrocyclotron | Synchrotron | |
---|---|---|---|
Magnetic field | Fixed | Fixed | Varying |
RF-frequency | Fixed | Varying | Varying |
Orbit size | Outward spiral | Outward spiral | Fixed |
Energy | Fixed | Fixed | Adjustable |
Operation | Continuous | Pulsed (fast) | Pulsed (slow) |
Acceleration stages | Single | Single | Multiple |
Ion source | Internal | Internal | External |
Vertical focusing | Spiral sector focusing | Weak | Strong |
Intensity control | Easier | Average | More difficult |
Footprint | Average | Smaller | Larger |
Weight | Larger | Smaller | Average |
Complexity | Easier | Average | More difficult |
Beam intensity | High | Average | Low |
Beam output emittance | Symmetrical | Symmetrical | Elliptical |
Power consumption | Average | Lowest | Highest |
Cost | Average | Lowest | Highest |
3.2 Proton Therapy: From Laboratory to Dedicated Medical Facility
The paper from 1946, written by Dr. Robert R. Wilson [14], contains the first information concerning the radiological uses of fast protons and created the future basis for proton therapy. The article discusses the need for accelerators producing proton beams with kinetic energies above 125 MeV and perhaps reaching as high as 400 MeV. Accelerators producing such high kinetic energies were very rare or still under development during that time. Most of these accelerators were based in research institutions and were cyclotrons and synchrocyclotrons.
Stimulated by the ideas of R. R. Wilson, the first experiments on biological tissue started in the early 1950s using the Berkeley 184-inch (4.67 m pole diameter) synchrocyclotron (Fig. 3.6) [15]. The development of this accelerator started in the early 1940s, and the first proton beam was obtained in 1948. The synchrocyclotron patent was filed in 1947 and granted in 1952 by McMillan [16], and the first patient was treated in 1954.