Traumatic brain injury is a common cause of death worldwide, affecting mostly young people. The most feasible examination to be performed in the emergency room is computed tomography. Magnetic resonance (MR) imaging is becoming a significant tool in the evaluation of severe brain trauma. Diffusion-weighted imaging is an advanced MR imaging sequence that has been used to detect areas of ischemia and tumor malignancy. This article describes the recent advances in trauma based on diffusion-weighted MR imaging findings and in brain injury from head trauma based on diffusion-weighted MR imaging and diffusion tensor MR imaging findings. Current and potential clinical applications are discussed.
Traumatic brain injury (TBI) is a common and devastating condition that exacts a heavy toll on the affected individuals, their families, and society in general. Worldwide, 10 million deaths and/or hospitalizations annually are directly attributable to TBI. It is reported that 1.4 million people incur TBI in the United States every year, but this statistic does not reflect the true incidence, because many individuals never seek medical attention. The most common causes of TBI are falls, motor vehicle accidents, sports-related injuries, and assaults.
Neuroimaging plays an important role in the evaluation of patients with TBI. Cranial computed tomography (CT) is the modality of choice for the initial assessment of moderate to severe TBI because it is fast, widely available, and highly accurate for the detection of skull fractures and intracranial lesions, which may necessitate immediate surgical intervention. Magnetic resonance (MR) imaging is the method of choice in patients with acute TBI when neurologic findings cannot be explained by CT. MR imaging is also recommended for the evaluation of TBI-related symptoms in the subacute or chronic phases of the injury. After the patient has been stabilized, imaging is directed at providing a clearer picture of the extent of injury and information regarding prognosis. The use of MR imaging is helpful to the physician, because it has a greater spatial resolution than CT, as well as an increased sensitivity to lesions within the brainstem, posterior fossa, and in regions adjacent to bone. Conventional MR imaging sequences usually provide valuable information related to the severity of the TBI, and are informative as to prognosis and the likelihood of long-term disabilities.
Advanced MR imaging techniques, such as diffusion-weighted imaging (DWI) and diffusion tensor imaging (DTI), have emerged as powerful noninvasive methods in neuroimaging, but the application of these techniques to TBI is recent. DWI describes an MR imaging sequence that is sensitive to the diffusion of water molecules within tissues. Restricted diffusion is expressed as a reduction in the apparent diffusion coefficient (ADC) and results in hyperintense signal intensities on DWI. Reduced diffusion is often interpreted as indicating cytotoxic edema, and is most frequently seen in ischemic stroke. DTI measures both the direction and magnitude of the water-associated proton diffusion. Diffusion in white matter is nonrandom, being primarily constrained to an axis parallel to axons, a property referred to as anisotropy. Changes in fractional anisotropy (FA) are interpreted as alterations in white matter ultrastructure. Both DWI and DTI can show abnormalities in the white matter in the acute and chronic phases after TBI. These findings are sometimes beneath the resolution of standard neuroimaging techniques, such as CT and conventional MR imaging. These measures are often directly applicable to the prognosis of the patient, predicting both the duration of coma and outcome in patients with severe TBI.
This article discusses both the current and potential clinical applications of DWI and DTI in patients with TBI.
Acute stage imaging
Acute Hematomas
Intracranial bleeding (intra-axial and extra-axial) are common acute findings after TBI. CT scans and conventional MR imaging sequences are the gold standard imaging techniques for the evaluation of these patients. However, DWI may help in some cases, because in the acute phase, a blood clot may appear with high signal intensity on DWI and low signal intensity on ADC, representing reduced diffusion. In the days after injury, a T2 shine-through effect can be seen and, within weeks, hematomas show facilitated diffusion ( Fig. 1 ). In addition, DWI may facilitate detection of small acute and subacute intraventricular hemorrhage, with accuracy equal to that of CT.
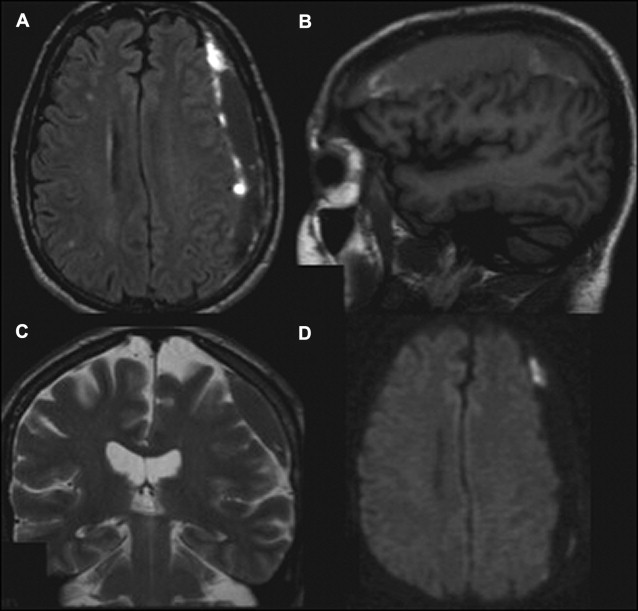
Diffuse Cerebral Swelling
Severe brain trauma (Glasgow Coma Scale ≤8) triggers a cascade of molecular and cellular events, culminating in a loss of ionic gradients in the brain. Water influx to the intracellular compartment leads to restricted water diffusion. This intracellular edema is cytotoxic, and is similar to that seen in ischemia. Head injury may also cause damage to the blood-brain barrier, usually around parenchymal lesions, increasing the extracellular water content and resulting in vasogenic edema. Edema itself constitutes a secondary insult resulting from trauma, which leads to intracranial hypertension and decreased cerebral perfusion, the main cause of morbidity and mortality after trauma. Intracranial hypertension also leads to increased edema, and can result in ischemic lesions. Thus, diffuse cerebral swelling is represented by an increase in tissue fluid and a combination of cytotoxic and vasogenic edema.
Areas of both cytotoxic and vasogenic edema have high signal intensities on T2-weighted images (T2-WI), and DWI plays a role in differentiating between these injury types. Cytotoxic edema presents with low ADC values (low signal intensity on ADC maps) and high signal intensities on DWI, reflecting restricted diffusion ( Fig. 2 ). These changes can be observed as soon as 8 hours after the injury, and are mainly related to diffuse axonal injury (DAI). Within the first 2 weeks after injury, in most cases the edema increases in severity, as the ADC decreases, and normalizes around the 10th day, similar to the pseudonormalization that occurs in ischemia. Animal models are consistent with these observations, showing that ADC values progressively decline after acute head injury, reaching the lowest values 1 to 2 weeks after injury. As seen in ischemic lesions, a penumbral area can be observed around the parenchymal traumatic lesion. The penumbra is represented by low ADC values that surround a region with even lower ADC values, and may represent an extension of the lesion. The similarities observed between ischemic and post-TBI lesions lend support to the idea that ischemia and edema are usually associated, as has been shown in animal models. Cytotoxic edema and ischemia are intrinsically correlated to such a high degree that an ischemic injury should be excluded with additional MR imaging sequences if the lesions with restricted diffusion are not located at the predilection sites of DAI.
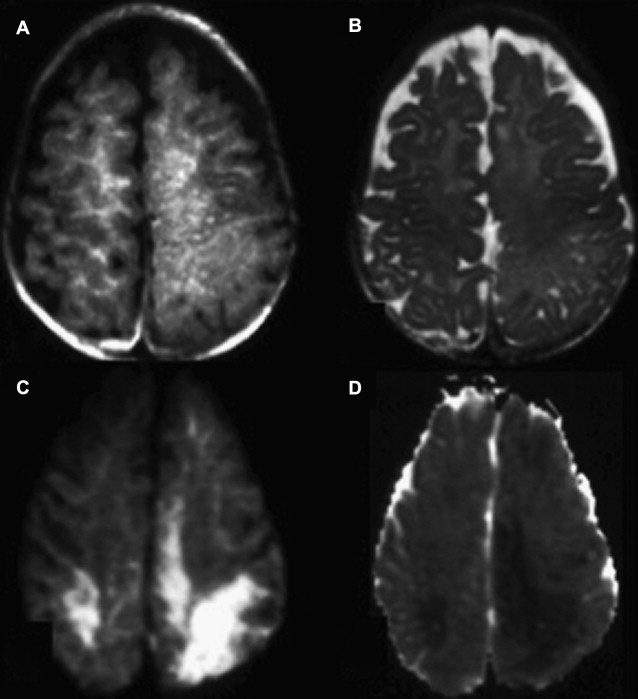
Cytotoxic edema that occurs without ischemia may be designated as neurotoxic edema. Neurotoxic edema may be instigated by a direct trauma mechanism. Trauma leads to a lesion of the axonal membrane, resulting in the leakage of glutamate into the extracellular space and excitotoxicity injury. High extracellular glutamate induces aberrant calcium and sodium influx into neural cells, resulting in the induction of apoptosis, gliosis, delayed neuronal death, and infarction. Excitotoxicity may be the underlying damage mechanism in the penumbral area surrounding a lesion after head injury.
Glial cells such as astrocytes and oligodendrocytes also express glutamate receptors. Alternatively, the intracellular edema may occur in these cells, thus protecting axons from increased glutamate uptake. Intramyelin and neurotoxic edema represent slower forms of cellular swelling and may be responsible for the extended time over which decreased ADC values are observed after TBI.
Vasogenic edema presents with different findings in MR imaging, displaying high signal intensities on the ADC maps and DWI (T2 shine-through effect) or, less commonly, high signals on ADC and low signals on DWI (facilitated diffusion). These changes in signal intensities are usually located around a focal parenchymal lesion, such as a hematoma, because the blood clot exerts an osmotic effect on the surrounding brain parenchyma. The clot is usually present after the injury and stable over the following weeks. These findings suggest that intracellular edema should be more relevant to the clinicians, because it increases over time, and is considered the major contributor to posttraumatic brain swelling in closed-head trauma.
DTI parameters may also be affected by brain swelling. FA reduction may occur as early as 24 hours after trauma, and last for at least 7 days. The entire white matter may show reductions in the FA during this acute phase, based on increased radial diffusivity values with no disturbance in the axial diffusivity. If the ADC values are also increased, vasogenic edema is indicated; this is a reversible and treatable damage mechanism.
Ischemia and Infarction
Hypoxic-ischemic injury is usually seen after TBI and it is related to poor clinical outcome. In most cases, this type of injury is caused by either local or systemic effects. Local factors can include cerebral edema with increased intracranial hypertension, as well as intracranial hematomas. These factors can lead to compression of intracranial blood vessels, thereby causing hypoperfusion and ischemia, which can involve a whole hemisphere. Vasospasms induced by subarachnoid hemorrhage may also play a role in cerebral infarction. Systemic factors like hypotension and hypoxia caused by the trauma, and distal embolization secondary to the vascular dissection or fat embolism, may be involved as well. Acute ischemic areas are easily observed using DWI, because they have reduced diffusion that presents with high signal intensities on DWI and low signal intensities on ADC maps.
Subacute stage imaging
DAI
DAI results from rotational acceleration and deceleration forces that produce diffuse shear-strain deformations of brain tissue. DAI usually takes place at the junction of the gray and white matter, particularly in the corpus callosum (CC), and at the dorsolateral aspect of the upper brain stem, because the border between these areas and the adjacent tissue differs in density and rigidity. As a result, disruptions in ionic homeostasis and altered permeabilities of the axolemma occur. Initially, damage manifests as the development of axonal bulbs, or retraction balls, with subsequent progression to microglial clusters and long-tract degeneration. Axonal disconnection may happen 30 to 60 hours after trauma, leaving both axonal segments with independent myelin sheaths. Axonal injury occurring at acute time points is followed by demyelination and edema at subacute time points. The force of impact causing the brain injury also determines the amount of fiber misalignment and/or disruption.
DWI is a sophisticated tool for the evaluation of DAI lesions, because it is more sensitive than T2-WI and fluid-attenuated inversion recovery (FLAIR) in the acute phase of injury after trauma. In addition, the lesion volume depicted with DWI has a stronger correlation with clinical outcome than that obtained from other conventional sequences. The high signal intensity lesions observed with DWI may have high or low ADC values, and this additional information allows the classification of the injury in terms of severity. ADC values significantly decrease in typical DAI lesions observed with conventional MR imaging, as early as 1 day after the head injury, and decreases persist up to 18 days ( Fig. 3 ). This finding is likely related to cytotoxic edema, which has previously been associated with DAI. Thus, lesions with low ADC indicate severe head injury and might be predictive of the patient’s long-term prognosis. DAI may also be represented as hemorrhagic lesions, which are better identified with T2*-WI. DWI may miss some of these lesions, because the effect of blood products on ADC is uncertain. Blood clots typically reduce the motion of water molecules. In addition, cytotoxic edema is usually present with such lesions. Hemorrhagic DAI may therefore present with low ADC values.
DAI lesions may be observed using DTI within the first few hours after head trauma. DTI is susceptible to changes in parenchymal structure that are caused by misalignment of fibers, edema, axonal degeneration, fiber disruption, and demyelination. Experiments with an animal model show that the initial days after trauma are characterized by axonal injury and resultant decreases in axial diffusion. After a week, axonal injury is followed by demyelination, and radial diffusion is then increased. Thus, the combination of reductions in axial diffusion values from axonal and cytoskeleton misalignment and increasing radial diffusion from demyelination, which favors diffusion in planes perpendicular to axons, leads to decreased FA values.
FA measurements may be assessed in normal-appearing white matter (NAWM) on T2-WI to detect DAI. The NAWM of trauma patients has been studied with DTI 1 year after the injury. In these patients, FA values were lower than the controls in the NAWM of the CC, the internal capsule, and the centrum semiovale, and were correlated with clinical outcome ( Fig. 4 ). The ADC values in the NAWM of the hemispheres and CC were higher in trauma patients than in controls, because of the loss of myelinated axon fibers secondary to the trauma.
The CC is more susceptible to DAI, because it is a less mobile structure connecting 2 mobile structures, the cerebral hemispheres. This characteristic is more important in the posterior portion of the CC, as indicated by a pathologic-anatomic study. The genu of the CC may be affected even in mild head trauma. FA decreases and ADC increases in the CC can be observed within the first 3 months after trauma, likely as a result of vasogenic edema, because these changes disappear after 3 months after injury. However, in moderate and severe head trauma, FA decreases in the splenium of the CC are not accompanied by ADC variations within the first 3 months. This finding suggests that lesions in the posterior part of the CC have diffusion patterns distinct from those in the genu, which may reflect more irreversible lesions. Lesions with low ADC, as a result of cytotoxic edema, are also frequently present in the CC during the acute phase of severe TBI ( Fig. 5 ).
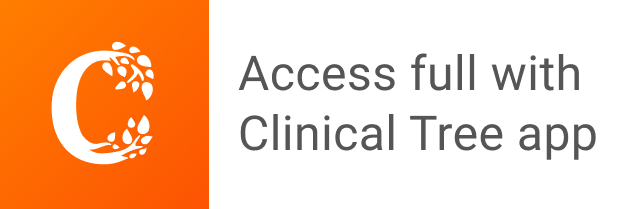