Essential Science of Nuclear Medicine
Ramsey D. Badawi
Linda A. Kroger
Jerrold T. Bushberg
Relevant Aspects of Radiation Physics
Types of Radiation in Nuclear Medicine. The electromagnetic spectrum of radiation can be divided into nonionizing and ionizing radiation. Nonionizing radiation includes commonly encountered forms of electromagnetic radiation such as visible light, microwave, and radiofrequencies (used in radio transmissions and in magnetic resonance imaging or MR). The ionizing radiation used in diagnostic medical imaging includes x-rays, γ-rays, and annihilation radiation. The difference between these forms of radiation lies in their origin. X-rays are extranuclear in origin and can be produced by bombarding an atom with photons or electrons; in diagnostic radiology, this is achieved with an x-ray tube, which produces x-rays with energies at and below 140 keV. γ-rays are produced from within the atomic nucleus as unstable nuclei transition to a more stable state. In diagnostic radiology, γ-ray energies lie typically in the 80 to 350 keV range (Table 53.1). Annihilation radiation is produced when a particle and its antiparticle interact and annihilate each other—this is the type of radiation detected in PET. When positrons annihilate, the resulting radiation has a fixed energy of 511 keV.
Ionizing radiation need not be part of the electromagnetic spectrum—it can also come in a particulate form. The particle of most common medical interest is the β-particle, which is an electron that has its origin within the unstable nucleus. As opposed to x-rays, γ-rays, or annihilation radiation, β-particles interact quite easily with matter, traveling only a few millimeters in tissue as they transfer their energy to their surroundings. This energy-transferring property produces a high dose within a short range and provides therapeutic usefulness in such entities as Graves disease and thyroid cancer with Iodine-131. β-antiparticles (β+) are the positrons used in PET imaging—they interact in and deliver radiation dose to human tissue in a similar way to ordinary β-particles prior to annihilating.
A form of radiation that is only rarely of interest for nuclear medicine imaging but which can be of importance when considering radiation safety is bremsstrahlung, or “braking radiation.” Bremsstrahlung can be generated when high-energy electrons or positrons interact with an atomic nucleus. Bremsstrahlung events become more likely as particle energy increases and as the effective atomic number of the nucleus increases. Surrounding the source with material such as plastic, which has a low effective atomic number, will stop the particles and minimize the radiation hazard. If necessary, any additional photon radiation can be reduced by surrounding the plastic shielding layer with a denser material such as lead. Occasionally, bremsstrahlung imaging with a gamma camera may be used to validate dose distribution after the administration of β-particle-emitting therapeutics such as yttrium-90-laden microspheres. The spatial resolution of the resulting images is very poor.
Photon Interactions With Matter. There are two primary interactions of photons with matter at the energies of interest for nuclear medicine. These are photoelectric interactions and Compton interactions. In a photoelectric interaction, a photon interacts with an atom and transfers all of its energy to an orbital electron (Fig. 53.1 right, photon interactions), disappearing in the process. The electron is ejected, ionizing the target atom. The ejected electron may have sufficient energy to subsequently ionize other atoms that it interacts with. In a Compton interaction, also known as a scattering event, the incident photon interacts with an atom and imparts part of its energy to an orbital electron. The electron recoils, and a new, lower energy (longer wavelength), photon is emitted at an angle to the incident photon trajectory (Fig. 53.1 left). This scattering angle is dependent upon the amount of energy lost to the electron. Small angles are associated with small transfers of energy to the electron, and the maximum scattering angle, 180 degrees, is associated with the maximum transfer of energy.
When a photon interacts with an atom, the relative likelihood of a photoelectric or a Compton interaction is dependent on the photon energy and on the density of the target material. For typical nuclear medicine photon energies in human tissue, the Compton scattering interaction is the most prevalent, becoming completely dominant at the higher end of the range (e.g., for Gallium-67 or PET imaging). However, the detector materials used for nuclear medicine are much denser than
tissue, and for these both photoelectric and Compton interactions are important.
tissue, and for these both photoelectric and Compton interactions are important.
Table 53.1 Radionuclides | ||||||||||||||||||||||||||||||||||||||||||||||||||||||||||||||||||||||||||||||||||||||||||||||||||||||||||||
---|---|---|---|---|---|---|---|---|---|---|---|---|---|---|---|---|---|---|---|---|---|---|---|---|---|---|---|---|---|---|---|---|---|---|---|---|---|---|---|---|---|---|---|---|---|---|---|---|---|---|---|---|---|---|---|---|---|---|---|---|---|---|---|---|---|---|---|---|---|---|---|---|---|---|---|---|---|---|---|---|---|---|---|---|---|---|---|---|---|---|---|---|---|---|---|---|---|---|---|---|---|---|---|---|---|---|---|---|
|
![]() Figure 53.1. Relevant Photon Interactions With Matter. Left: Photoelectric interaction. Right: Compton scatter. |
Units. Various units to describe radiation and its effects have been established. Because the scientific community in the U.S. is in transition between conventional units and the Système Internationale (SI), a table of both units with their conversion factors is provided in Table 53.2. Activity is used to describe the quantity of the radionuclide being administered and represents the rate of nuclear transformations, denoted by Curies (Ci) in conventional units and Becquerels (Bq) in SI units. The Roentgen is the unit utilized to express radiation exposure and is a measure of the ability of x-rays and γ-rays to produce a given amount of ionization in a given volume of air (Coulombs per Kilogram in SI units). From a biologic point of view, the important consideration is how much of the radiation exposure is deposited in an individual at a particular location. Absorbed dose is the amount of energy deposited by ionizing radiation per unit mass in joules/kg; the conventional unit is the radiation absorbed dose (rad) and the SI unit is the Gray (Gy). Because certain types of radiation are more biologically damaging than others, a radiation weighting factor (also called quality factor) is multiplied by the absorbed dose to yield the equivalent dose, which is measured in rem (roentgen equivalent man) in conventional units and the Sievert (Sv) in SI units. The equivalent dose unit allows comparison between the various ionizing radiation sources (photons, β-particles, α-particles). Because the quality factor is equal to one for photons and electrons, one roentgen approximately equals one rad in the diagnostic energy range for soft tissue, which approximately equals one rem (Fig. 53.2). That is, 1 R ≈ 1 rad (0.01 Gy) ≈ 1 rem (0.01 Sv).
Table 53.2 Conventional and Si Radiologic Units and Conversion Factors | |||||||||||||||||||||||||||||||||||||||||||||||||||||||||
---|---|---|---|---|---|---|---|---|---|---|---|---|---|---|---|---|---|---|---|---|---|---|---|---|---|---|---|---|---|---|---|---|---|---|---|---|---|---|---|---|---|---|---|---|---|---|---|---|---|---|---|---|---|---|---|---|---|
|
In recognition of the different sensitivity of various tissues and organs to radiation induced damage, the mean equivalent doses (HT), for specified tissues and organs of the body are modified by a dimensionless tissue weighting factor (WT) that takes into account the relative radiation detriment for the tissue or organ. This quantity is the effective dosea (E), thus E = ΣWT × HT, where ΣWT = 1. The primary detriment is the probability of fatal cancers however other factors such as the probability of severe heritable effects in future generations and other considerations are also included. The tissue weighting factor for a particular tissue or organ represents the fraction of the total radiation detriment to the whole body attributed to that tissue when the whole body is irradiated uniformly. The tissue weighting factors have been developed from a reference population of equal numbers of both sexes and a wide range of ages and, as such, should not be used to calculate the dose to a specific patient for the purpose of assigning risk. The WT values are: 0.01 for bone surface, brain, salivary gland and skin; 0.04 for bladder,
esophagus, liver and thyroid; 0.08 for gonadsb; 0.12 for breast, bone marrow, colon, lung, stomach, and the remainder tissuesc.
esophagus, liver and thyroid; 0.08 for gonadsb; 0.12 for breast, bone marrow, colon, lung, stomach, and the remainder tissuesc.
Radiation Safety
Radiation Exposure to the Worker. The total average exposure for an x-ray technician results in an annual equivalent dose of only 0.5 to 1 Sv (50 to 100 mrem) while for the nuclear medicine technologist it is 2 to 3 Sv (200 to 300 mrem). Occupational exposure to nuclear medicine technologists performing PET procedures can be four to five times higher due to the high-energy gamma rays. The majority of whole-body radiation to the nuclear medicine worker comes from exposure to the patient during dose administration and while setting the patient up for imaging. Localized extremity exposure doses from radiopharmaceutical preparation and injection can be higher.
The Nuclear Regulatory Commission (NRC) limits for radiation exposure (total effective equivalent dose) are 50 mSv/y (5 rem/y) for designated occupationally exposed workers and 1 mSv/y (100 mrem/y) for members of the public.
The primary risk from any increased radiation exposure is an increased risk for cancer. For each additional Sievert effective dose, the lifetime increased risk of cancer is approximately 5% (5 × 10-4 per rem). The cancer risk in the general population is 41%. Therefore, a lifetime occupational whole-body dose of 50 mSv (5 rem) – e.g. 2.5 mSv/y × 20 years – will increase the risk of developing cancer from 41 to 41.25%. With these numbers, several caveats to radiation exposure need to be remembered: any increased risk is spread over a lifetime; increased risks from exposure are additive; and the minimum latency of cancer is 4 to 8 years with the mean for solid tissue tumors being closer to 20 to 25 years.
Because contamination in the workplace also increases radiation exposure to the worker, several guidelines can be followed to minimize contamination: (1) follow standard precautions by wearing protective clothing; (2) use plastic-backed absorbent to restrict any spills; (3) wash hands frequently; (4) use covers over collimators that can be discarded if contaminated; (5) monitor and wipe test frequently (see below); and (6) avoid eating, or drinking when handling radioactivity.
Radiation Exposure to the Patient. The average annual per capita effective dose to the population in the United States is 6.2 mSv/y (620 mrem/y). Natural background radiation from atmospheric and terrestrial sources contributes 3.1 mSv/y (310 mrem/y) (∼50%) while man-made sources, almost entirely from medical procedures, provide the other 3.1 mSv/y (∼50%). Nuclear medicine examinations account for ∼26% of the dose from medical procedures with the largest contribution coming from Tc-99m and Tl-201 myocardial perfusion imaging.
Because nuclear medicine procedures involve radionuclides and radiopharmaceuticals that are variably transient in the human body, dosimetric considerations include not only the initial activity administered but the biodistribution, the physical and biologic half-lives, as well as possible pathologic processes. The physical half-life is defined as the time required for the number of radioactive atoms in a sample to decrease by one-half, while the biologic half-life is the time required for half of the radionuclide to be eliminated by biological processes. On a relative scale, the dose to the whole body from most nuclear medicine diagnostic procedures is equivalent to one-half to six times the average annual per capita effective dose from natural background. That is, the effective dose for nuclear medicine examinations range from 1.5 mSv for a Tc-99m MAA lung perfusion study to 20 mSv for a tumor imaging study with 185 MBq of Ga-67 citrate. Additional dose might be received during a PET study from the x-ray component of the CT
as patients are typically scanned on a combined PET-CT or SPECT-CT device. There are no standard scanner parameters for the CT component of a PET-CT or SPECT-CT procedure, so this additional dose can vary widely.
as patients are typically scanned on a combined PET-CT or SPECT-CT device. There are no standard scanner parameters for the CT component of a PET-CT or SPECT-CT procedure, so this additional dose can vary widely.
Table 53.3 Recommendations for Cessation of Breast Feeding After Administration of Radiopharmaceuticals to Mothers | ||||||||||||||||||||||||||||||||||||||||||||||||||||||||||||||||||||||||
---|---|---|---|---|---|---|---|---|---|---|---|---|---|---|---|---|---|---|---|---|---|---|---|---|---|---|---|---|---|---|---|---|---|---|---|---|---|---|---|---|---|---|---|---|---|---|---|---|---|---|---|---|---|---|---|---|---|---|---|---|---|---|---|---|---|---|---|---|---|---|---|---|
|
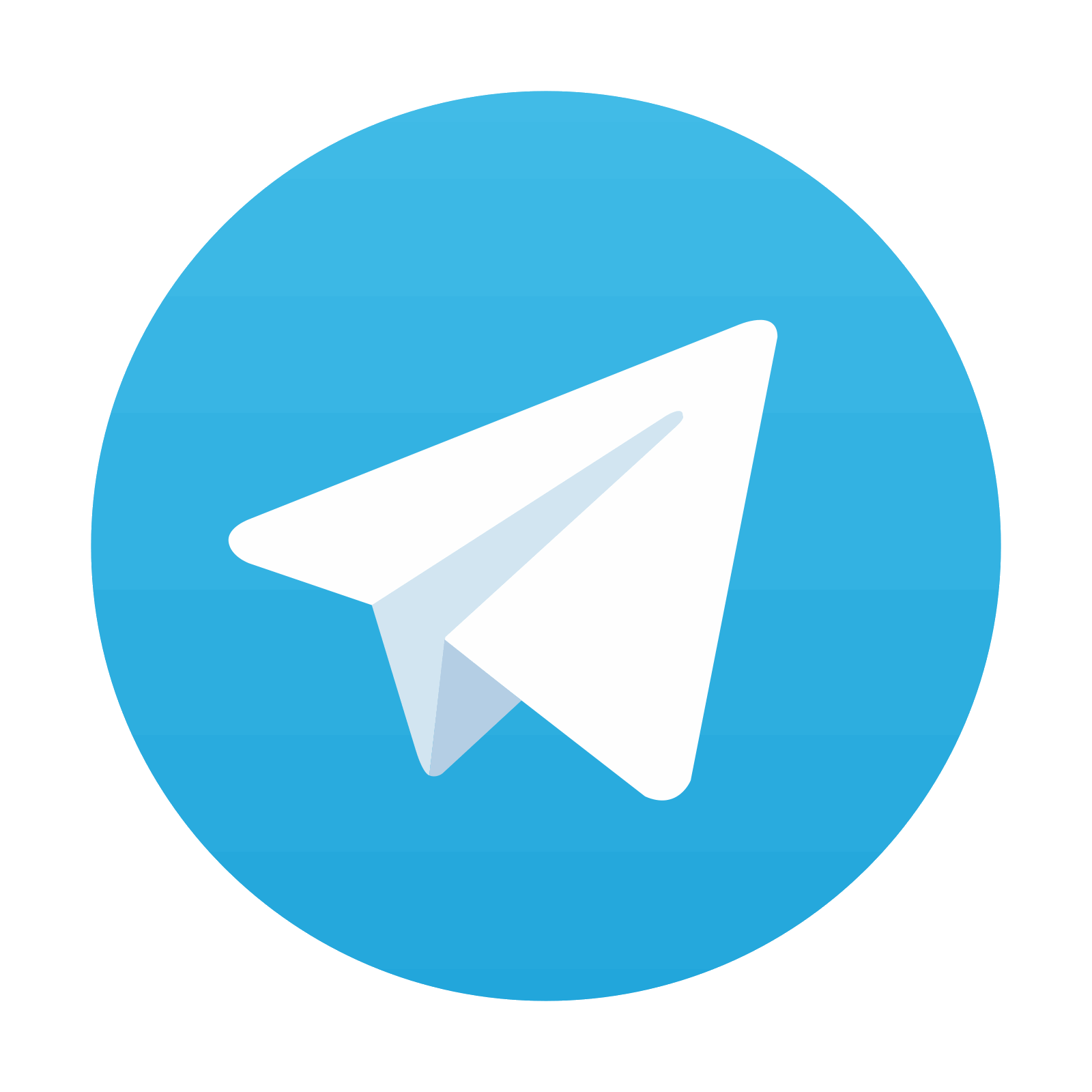
Stay updated, free articles. Join our Telegram channel
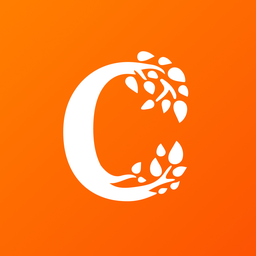
Full access? Get Clinical Tree
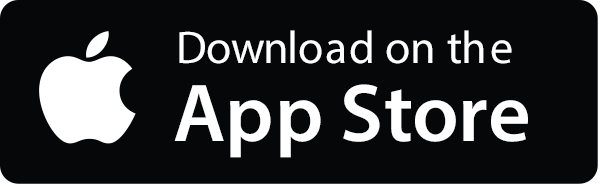
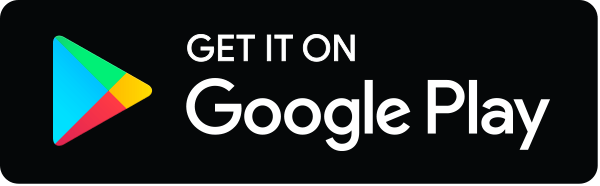