Fig. 58.1
Coronal maximum intensity projection (MIP) image of a normal F-18 FDG PET in an 11-year-old. FDG uptake is seen in tonsillar tissue (black arrow) and homogenously throughout the thymus (arrow heads). Symmetric uptake is also present in the metabolically active (brown) supraclavicular fat (white arrow)
58.2 Other Nuclear Medicine Studies
Many of the techniques described to prepare children for PET/CT imaging, including the explanation of the procedure, immobilization, and obtaining IV access, are similar for other nuclear medicine studies. As with FDG PET, pediatric nuclear medicine studies differ from similar adult studies in radiopharmaceutical dosing [4]. The Pediatric Nuclear Medicine Dose Reduction Workgroup developed dosing guidelines for nine commonly used radiopharmaceuticals in response to a 2008 survey of 13 leading pediatric radiology departments that showed considerable variation in radiopharmaceutical doses [4, 5] (Table 58.1).
Table 58.1
North American Consensus Guidelines for Administered Radiopharmaceutical Activities in Children and Adolescentsa
Radiopharmaceutical | Recommended administered activity (based on weight only) | Minimum administered activity | Maximum administered activity | Comments |
---|---|---|---|---|
123I-MIBG | 5.2 MBq/kg (0.14 mCi/kg) | 37 MBq (1.0 mCi) | 370 MBq (10.0 mCi) | |
99mTc-MDP | 9.3 MBq/kg (0.25 mCi/kg) | 37 MBq (1.0 mCi) | ||
18F-FDG | Body | 37 MBq (1.0 mCi) | Low end of dose range should be considered for smaller patients. Administered activity may take into account patient mass and time available on PET scanner | |
3.7–5.2 MBq/kg (0.10–0.14 mCi/kg) | ||||
Brain | ||||
3.7 MBq/kg (0.10 mCi/kg) | ||||
99mTc-DMSA | 1.85 MBq/kg (0.05 mCi/kg) | 18.5 MBq (0.5 mCi) | ||
99mTc-MAG3 | Without flow study | 37 MBq (1.0 mCi) | 148 MBq (4 mCi) | Administered activities at left assume that image data are reframed at 1 min/image. |
3.7 MBq/kg (0.10 mCi/kg) | ||||
With flow study | Administered activity may be reduced if image data are reframed at longer time per image | |||
5.55 MBq/kg (0.15 mCi/kg) | ||||
99mTc-IDA | 1.85 MBq/kg (0.05 mCi/kg) | 18.5 MBq (0.5 mCi) | Higher administered activity of 37 MBq (1 mCi) may be considered for neonatal jaundice | |
99mTc-MAA | If 99mTc used for ventilation | 14.8 MBq (0.4 mCi) | ||
2.59 MBq/kg (0.07 mCi/kg) | ||||
14.8 MBq (0.4 mCi) | ||||
No 99mTc ventilation study | ||||
1.11 MBq/kg (0.03 mCi/kg) | ||||
99mTc-pertechnetate (Meckel diverticulum imaging | 1.85 MBq/kg (0.05 mCi/kg) | 9.25 MBq (0.25 mCi) | ||
18F-sodium fluoride | 2.22 MBq/kg (0.06 mCi/kg) | 18.5 MBq (0.5 mCi) | ||
99mTc (for cystography) | No weight-based dose | No more than 37 MBq (1.0 mCi) for each bladder-filling cycle | 99mTc-sulfur colloid, 99mTc-pertechnetate, 99mTc-diethylene triamine pentaacetic acid, or possibly other 99mTc radiopharmaceuticals may be used. There is wide variety of acceptable administration techniques for 99mTc, many of which will work well with lower administered activities | |
99mTc-sulfur colloid (for oral liquid gastric emptying) | No weight-based dose | 9.25 MBq (0.25 mCi) | 37 MBq (1.0 mCi) | Administered activity will depend on age of child, volume to be fed to child, and time per frame used for imaging |
99mTc-sulfur colloid (for solid gastric emptying) | No weight-based dose | 9.25 MBq (0.25 mCi) | 18.5 MBq (0.5 mCi) | 99mTc-sulfur colloid is usually used to label egg |
58.3 Magnetic Resonance Imaging
Considerations in preparing children for nuclear medicine studies also apply to magnetic resonance imaging (MRI). Demonstration (often by child life personnel) is used to help prepare children for MRI more than any other modality in pediatric imaging. The long scan times, relatively small scanner bore, and loud noises make it a difficult study for patients to undergo without sedation. One way that child life specialists can demonstrate an MRI is through the use of a mock scanner (a scale or full-size model of the clinical scanners) [6–11]. In hospitals that provide this experience, the patient is often also able to take a tour of the radiology department, see a life-sized scanner, and hear the noises that the MRI will make during scanning.
In order to obtain high-quality MR images, the patient must remain still for the duration of the study. The strategy used to help children achieve this depends on the patient’s age. Neonates can often be imaged without sedation if they are fed and swaddled immediately prior to imaging. Infants and children between 3 months of age and 7 years often have difficulty lying still and are typically sedated or undergo general anesthesia to allow imaging. Older children and adolescents can be distracted through the use of MR-safe video goggles [12].
58.4 Leukemia and Lymphoma
Leukemia and lymphoma are often mentioned together because of the similarity of imaging findings of the two entities; however, the clinical manifestations of the two diseases vary considerably. Leukemia is the most common childhood malignancy, accounting for 25–33 % of pediatric cancers [13]. It can be classified as either acute or chronic and as arising from either a lymphoblastic or myeloid cell line. Acute lymphoblastic leukemia (ALL) accounts for 75 % of all pediatric leukemia [13].
More than a third of children with leukemia present with a limp or bone pain [13]. Functional imaging is not commonly utilized in the setting of leukemia. When functional imaging is used, the most commonly performed study is a bone scan using technetium (Tc)-99m methylene diphosphonate (MDP). There are four common patterns of abnormal uptake on a bone scan including symmetric increased uptake in the metadiaphysis of the lower limbs, diffuse increased skeletal uptake (superscan pattern), focal areas of increased uptake at sites of bone destruction or fracture, and focal decreased uptake at sites of osteonecrosis [13]. PET/CT is not commonly used to image patients with leukemia, although it may be performed in patients with extramedullary disease. In these patients, there is increased FDG uptake within the involved organs and within leukemic masses (chloromas), if present (Fig. 58.2).
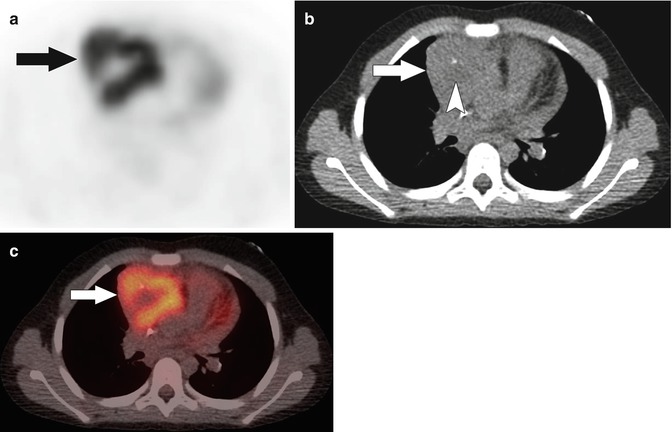
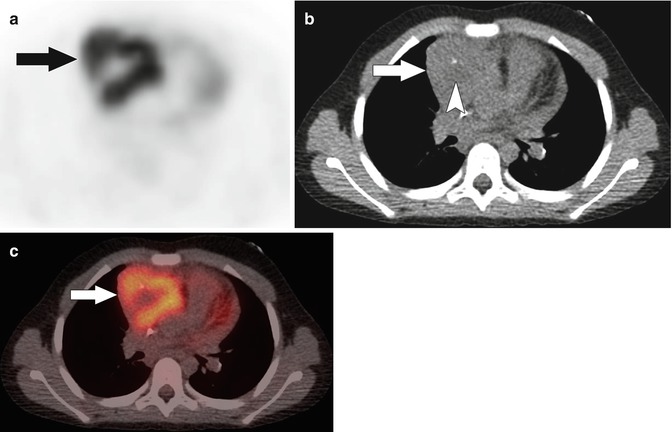
Fig. 58.2
(a) Axial F-18 FDG PET, (b) noncontrast localization CT, and (c) fused PET/CT images in a 7-year-old with acute myelogenous leukemia show moderate FDG uptake in an anterior mediastinal chloroma (arrows). Centrally within the mass there is an area of relatively lower FDG uptake and subtle low attenuation on CT (arrowhead in b) suggesting necrosis
Lymphoma is the third most common pediatric malignancy, typically occurring in older children and adolescents [13]. It is more common in males up to 15 years of age, at which time the male/female incidence becomes equal [13]. Lymphoma is separated into two main categories: Hodgkin lymphoma and non-Hodgkin lymphoma (NHL). Each is further broken down into subtypes. The nodular sclerosing subtype of Hodgkin lymphoma is the most common, accounting for 85 % of pediatric Hodgkin lymphoma [13].
Functional imaging, particularly PET/CT, plays a large role in the diagnosis, staging, and follow-up of pediatric patients with lymphoma. There have been multiple studies evaluating the role of PET/CT in adult lymphoma patients. These studies have shown that PET/CT is highly sensitive and specific for staging lymphoma (Fig. 58.3) [14–18]. Additionally, compared to conventional imaging, PET/CT is better able to distinguish between viable tumor and residual inflammatory or fibrous tissue [14, 19–21]. PET/CT is also able to provide prognostic information in terms of progression-free survival [14, 22, 23]. Many of these studies included adolescent patients so it is possible that the results are generalizable to pediatric populations.
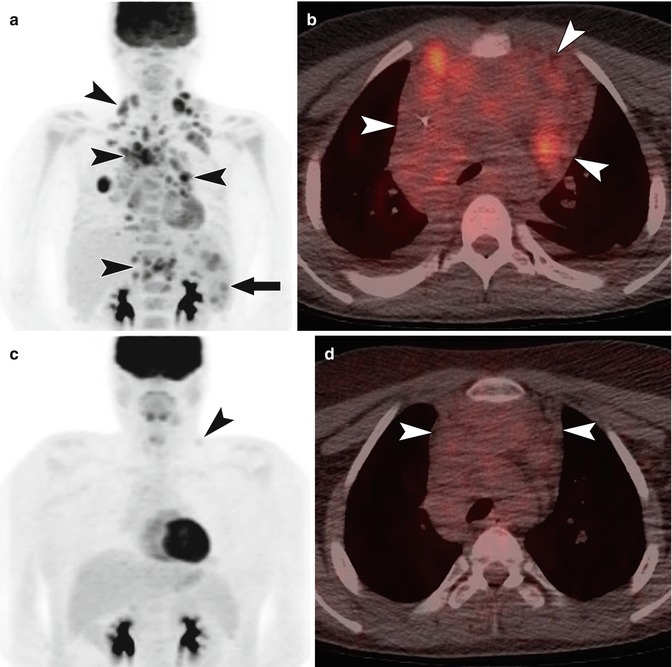
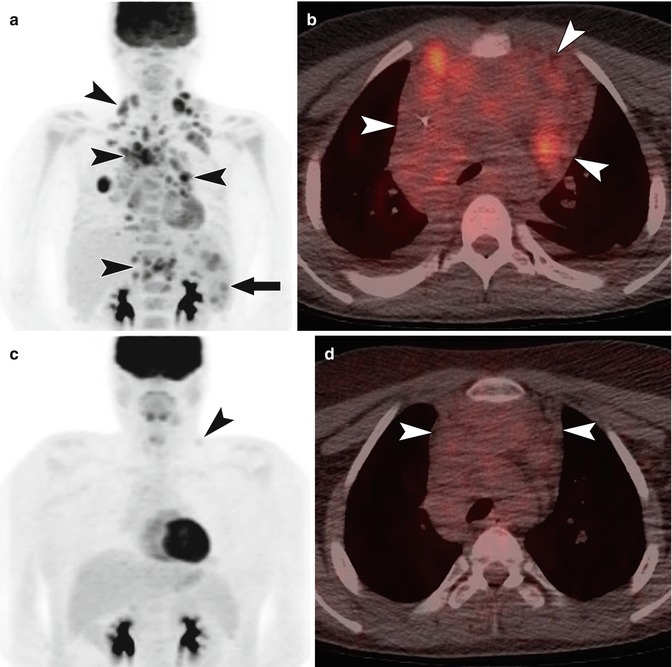
Fig. 58.3
A 15-year-old with classic Hodgkin lymphoma. (a) Coronal MIP image from F-18 FDG PET at the time of diagnosis shows FDG-avid disease involving cervical, supraclavicular, mediastinal, hilar, and upper abdominal lymph nodes (arrow heads). Nodular disease is also present in the spleen (arrow). Diffusely increased activity in the bone marrow reflects hematopoietic stimulation. (b) Fused axial image from an F-18 FDG PET/CT at the time of diagnosis shows FDG uptake within bulky, coalescent anterior mediastinal nodal disease (arrow heads). (c) Coronal MIP image from F-18 FDG PET in the same patient 5 weeks after initiation of therapy shows near complete response with a faint residual focus of activity in the left supraclavicular region (arrow head). (d) The fused axial image from an F-18 FDG PET/CT 5 weeks after initiation of therapy shows persistent bulky nodal disease in the anterior mediastinum (arrow heads) but without FDG uptake above mediastinal blood pool. Given the lack of abnormal FDG uptake, the persistently enlarged lymph nodes can be attributed to residual inflammatory/fibrous tissue
There are fewer studies specifically concerning the use of PET/CT in the diagnosis and staging of pediatric lymphoma. Available studies have shown that PET/CT is accurate and contributes to the clinical management of these patients [24–32]. London et al. compared the diagnostic accuracy of PET/CT to other conventional imaging modalities and found that PET/CT performed significantly better than conventional imaging with a sensitivity and specificity of 95.9 and 99.7 % (vs. 70.1 and 99 %) [24]. When the modalities were discordant, PET/CT was correct 86 % of the time [24]. PET/CT has also been shown to better depict additional sites of disease compared to contrast-enhanced CT resulting in upstaging of the tumor in nearly 15 % of patients [33]. In addition to its value in diagnosis and staging, PET/CT is more accurate than conventional imaging in predicting response to standard therapy [24]. Poor responders have persistent FDG uptake greater than mediastinal blood pool activity at sites of disease after two cycles of chemotherapy [24].
Bone marrow involvement is a sign of advanced lymphoma and its presence confers a less favorable prognosis [34]. Traditionally, marrow involvement was diagnosed via biopsy, but biopsy is an invasive procedure and only samples a fraction of a patient’s bone marrow [34]. Multiple studies in pediatric patients with Hodgkin lymphoma [34, 35] have shown that PET/CT is a highly sensitive, specific, and accurate method of detecting bone marrow involvement, comparable to bone marrow biopsy. One study found a considerably higher incidence of bone marrow involvement via PET/CT (25.7 %) as compared to bone marrow biopsy (4 %) [35]. Because PET/CT evaluates the entire body, it is able to identify focal marrow involvement distant from the typical biopsy location (Fig. 58.4).
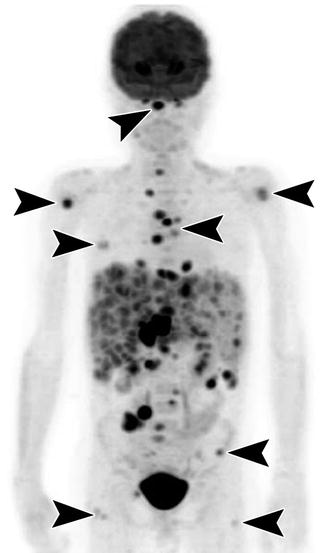
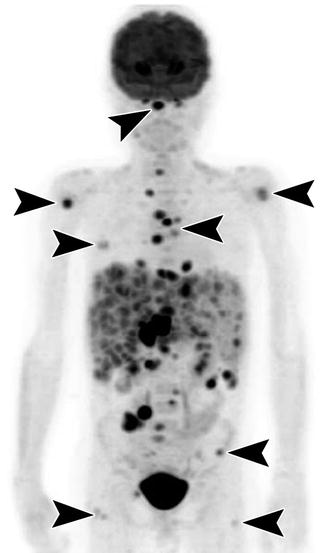
Fig. 58.4
Coronal MIP image from an F-18 FDG PET in a 10-year-old with diffuse large B-cell lymphoma shows extensive FDG-avid disease in the liver and spleen, which are enlarged, as well as within peritoneal and retroperitoneal lymph nodes. There are additionally nodular foci of FDG uptake in the bone at multiple locations (arrow heads) including the clivus, proximal humeri, ribs, spine, sternum, iliac wings, and proximal femurs. No FDG-avid disease is present in the medial iliac bones at the typical location of bone marrow biopsy
Patients diagnosed with lymphoma typically undergo multiple scans during diagnosis and therapy. Due to the radiation dose associated with PET/CT and the potential increased risk of malignancy after childhood exposure to radiation, there has been a drive to find a functional imaging modality that is radiation free. Diffusion-weighted imaging (DWI) and diffusion-weighted whole-body imaging with background body signal suppression (DWIBS) are MRI sequences that assess the movement of water molecules within tissue. DWI images are conventionally displayed white on black, while DWIBS is displayed black on white. DWIBS images are made to look like PET images and, like PET images, can be fused to anatomic images. Highly cellular tumors like lymphoma restrict the movement of water and appear bright on DWI and dark on DWIBS sequences. The apparent diffusion coefficient (ADC), calculated from diffusion data, can be used to quantify the degree of diffusion restriction in sampled pixels.
As DWI and DWIBS are emerging techniques in pediatric lymphoma imaging, there are few studies evaluating the efficacy of the technique and comparing it to other modalities. One study compared the mean ADC value on DWI to the maximum SUV on PET/CT [36] and found a strong inverse correlation between the two values at diagnosis. Low mean ADC values were associated with higher maximum SUV. These results are similar to findings in adults and suggest that MRI may ultimately be able to provide a sensitive, radiation-free method of detecting and staging pediatric lymphoma.
58.5 Brain Tumors
Tumors of the central nervous system (CNS) are the second most common tumors of childhood, representing 20 % of all pediatric malignancies [1]. The most common CNS tumors are medulloblastoma, juvenile pilocytic astrocytoma, ependymoma, and brain stem gliomas [1]. While conventional, anatomic imaging plays a primary role in diagnosing tumors of the central nervous system, functional imaging is used to diagnose tumors, plan therapy, determine prognosis, distinguish posttreatment effect from tumor, and detect recurrence.
While FDG is the preferred radiopharmaceutical for most oncologic imaging, it has limitations in neuro-oncology. Normal uptake of FDG in cortical gray matter due to baseline glucose metabolism results in low tumor-to-background uptake ratios [37]. FDG PET imaging of the brain can also be confounded by corticosteroids which are often used to treat patients with CNS neoplasms [37]. Corticosteroids alter systemic glucose metabolism resulting in elevated serum glucose levels and decreased cerebral uptake of glucose. Despite these difficulties, there have been several studies evaluating the use of FDG PET at the time of diagnosis in patients with central nervous system tumors. These studies have shown that increased FDG uptake is associated with more malignant tumors and poor survival [38–40] (Fig. 58.5). FDG PET also has value in the post-therapy patient to detect recurrent disease (increased FDG uptake) and distinguish it from both pseudoprogression and radiation necrosis (low or absent FDG uptake) (Fig. 58.6) [41]. While sensitive for recurrent disease, the specificity of FDG PET is somewhat lacking [41].
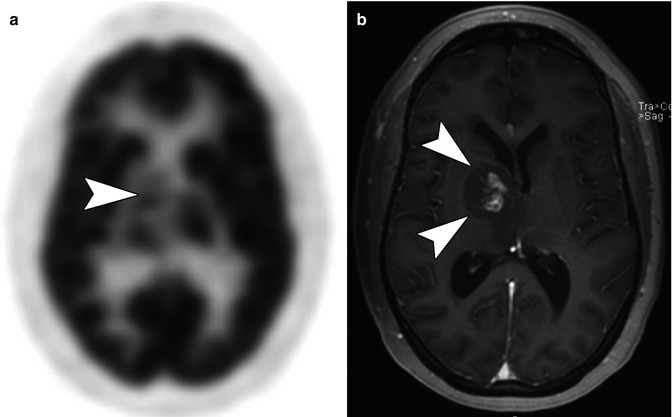
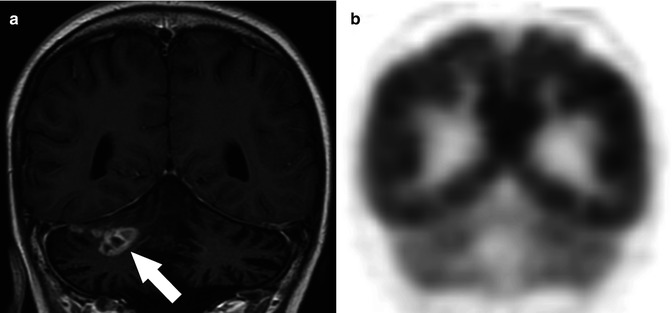
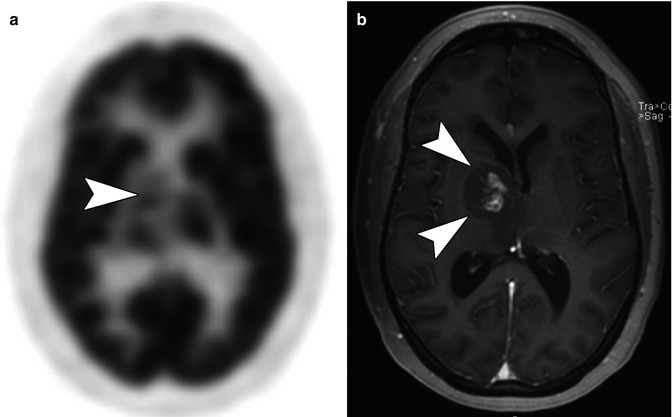
Fig. 58.5
(a) Axial F-18 FDG PET image in a 16-year-old with a right thalamic glioma shows FDG avidity in the right thalamus that is greater than surrounding white matter but less than normal cortical gray matter (arrow head). (b) Axial T1-weighted MR image performed after the administration of contrast shows central enhancement within the right thalamic tumor (arrow heads) corresponding to the area of increased FDG uptake seen FDG PET
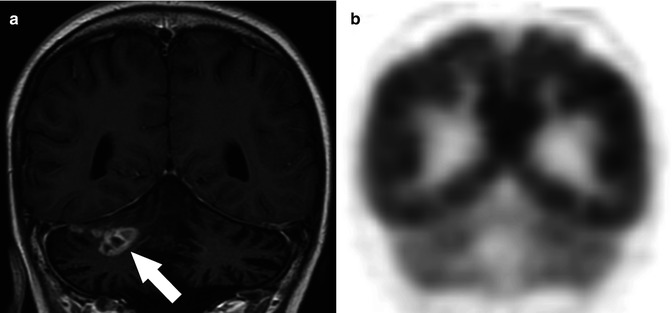
Fig. 58.6
(a) Coronal T1-weighted MR image obtained after the administration of contrast in an 18-year-old with a history of an anaplastic astrocytoma of the cerebellum status postradiation therapy shows an area of abnormal enhancement in the right cerebellar hemisphere (arrow) that was suspicious for residual tumor. (b) Coronal F-18 FDG PET at the corresponding location shows no abnormal FDG uptake in the lesion consistent with radiation necrosis
Other PET radiopharmaceuticals have been developed to overcome some of the limitations of FDG PET in neuroimaging. These include, but are not limited to F-18Fluorothymidine (FLT), C-11 methyl-l-methionine (MET), F-18Fluoroethyl-l-tyrosine (FET), and F-18Fluoro-l-dihydroxyphenylalanine. FLT and MET have received the most attention and are briefly reviewed here. None of these agents have been extensively studied in pediatric patients.
F-18Fluorothymidine is a thymidine analog that is used as a marker of tumor proliferation [37]. Background activity of FLT in the brain is very low, making the distinction of tumor from normal brain easier. There have been few studies evaluating the use of F-18FLT in children. These studies have shown that FLT may be useful in distinguishing tumor from infection, differentiating tumor from postradiation effects, and in characterization of tumors [42]. Further studies are needed to confirm these initial findings.
C-11 methyl-l-methionine is a radiolabelled amino acid that takes advantage of increased utilization of amino acids by brain tumors [43]. Similar to FLT, background activity of C-11 MET in normal brain is low, improving detection of tumor (Fig. 58.7). C-11 MET has been shown to be useful in determining the extent of tumors, in assessing the efficacy of treatment, and in identifying tumor recurrence [1, 44]. The main disadvantage of using C-11 radiopharmaceuticals is their short half-life of 20 min. Because of this, these radiopharmaceuticals have to be produced locally, limiting their use to sites with a nearby cyclotron.
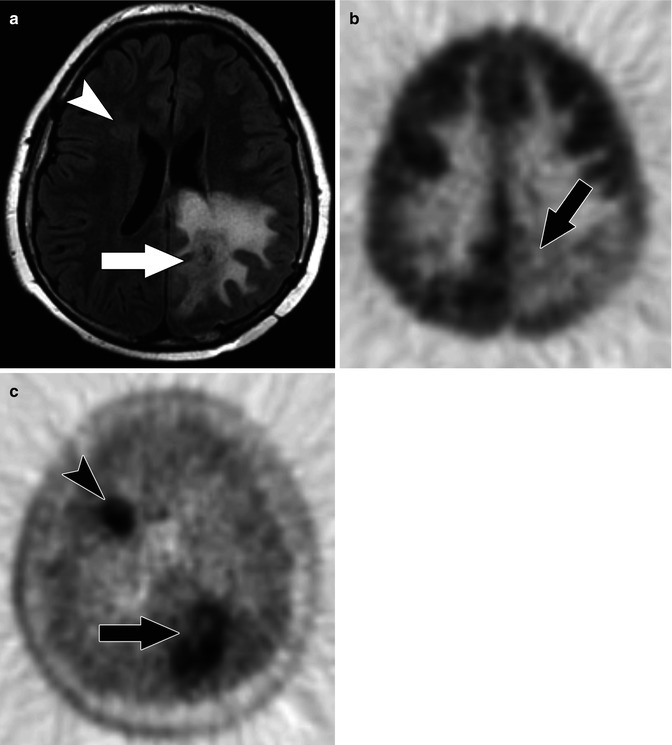
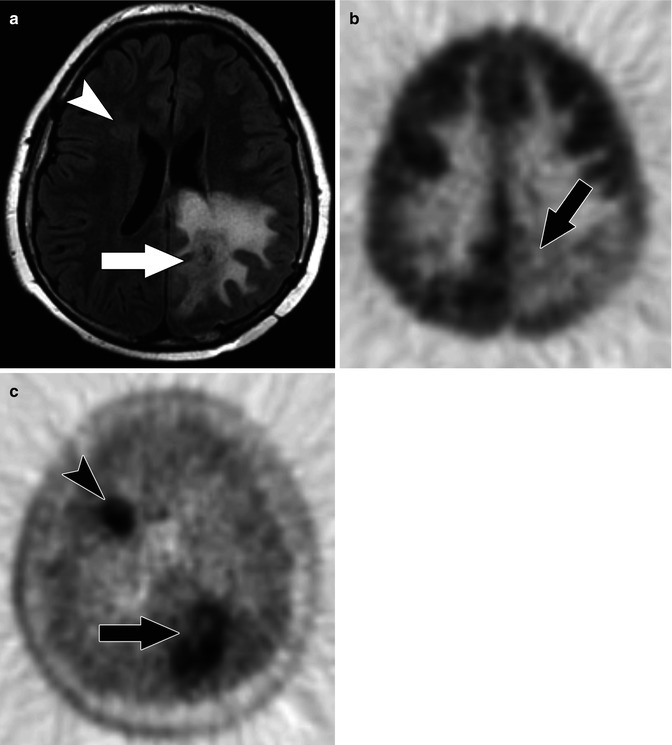
Fig. 58.7
(a) Axial FLAIR MR image in a 13-year-old with multifocal glioblastoma multiforme shows a dominant lesion in the left posterior parietal lobe with substantial surrounding edema (arrow). A more subtle area of increased FLAIR signal is present adjacent to the frontal horn of the right lateral ventricle (arrow head). (b) Axial F-18 FDG PET image shows relatively decreased uptake in the left posterior parietal lobe due to the edema and only faint uptake within the tumor (arrow). There is no abnormal FDG uptake related to the right frontal focus. (c) Axial C-11 MET PET image shows abnormal uptake of tracer in both the left parietal (arrow) and right frontal lesions (arrow head) reflecting multifocal tumor
In addition to PET imaging, planar and single-photon emission computed tomography (SPECT) imaging has been used to image brain tumors. Radiopharmaceuticals such as 99mTc glucoheptonate (GHA), 99mTc hexis-2-methoxyisobutylisonitrile (MIBI), 99mTc hexamethylpropyleneamine oxime (HMPAO), indium-111 pentetreotide, thallium-201, and 123I alpha-methyl tyrosine have all been used to differentiate pediatric brain tumors from post-therapy effects [1, 41, 45–47]. Of these tracers, thallium-201 has the advantage of absence of uptake in normal brain but is limited by low photon energy. Planar and SPECT imaging of brain tumors has fallen out of favor due to the emergence of modalities that combine high-quality morphologic images with functional data.
MRI plays a substantial role in the assessment of pediatric brain tumors. Of the routinely acquired sequences, DWI can be used as a measure of tumor cellularity with more cellular tumors showing restricted diffusion (Fig. 58.8) [37]. Like in body imaging, ADC values can be used to quantitate the degree of diffusion restriction; smaller ADC values are seen in the most cellular portions of a tumor. ADC values have also been used to assess a tumor’s response to therapy [37].
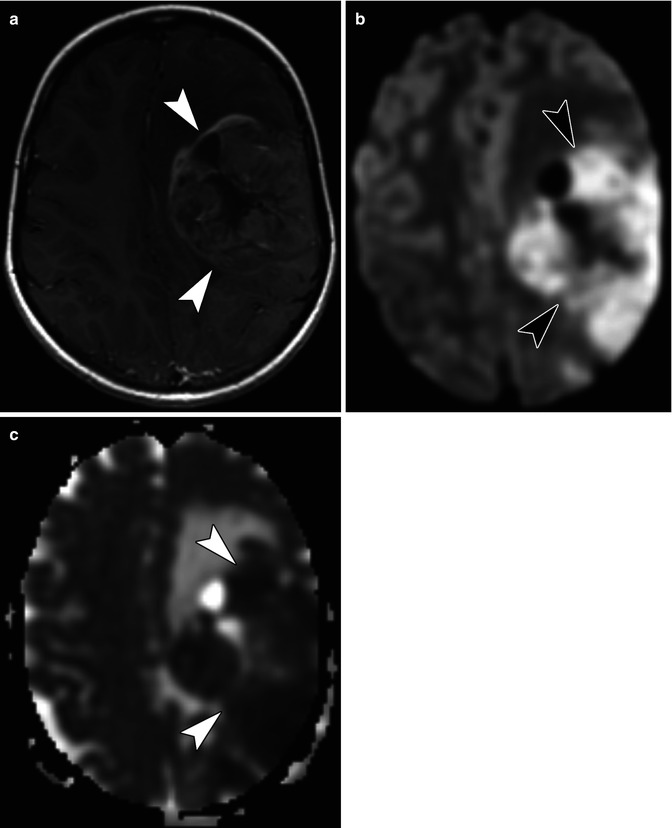
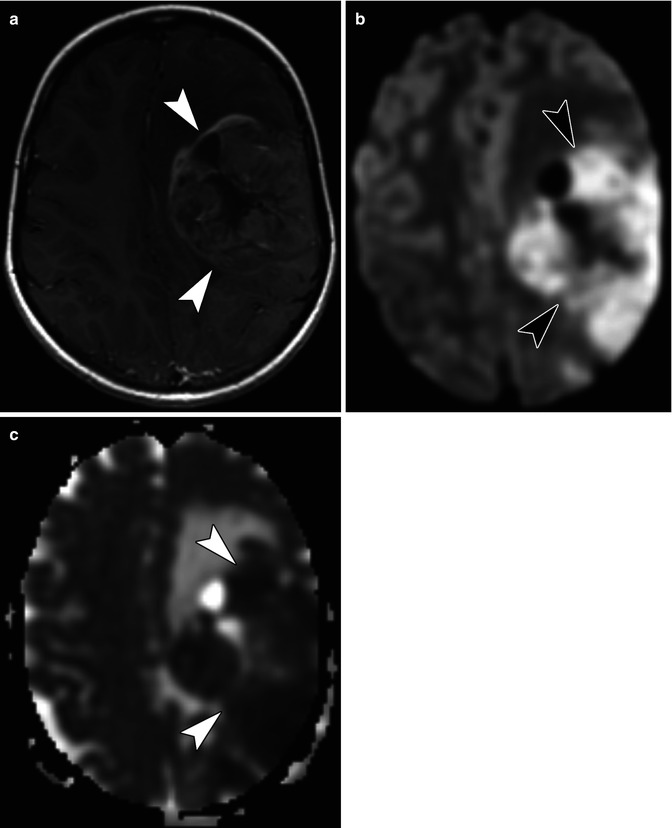
Fig. 58.8
(a) Axial T1-weighted post-contrast MR image in a 7-year-old with an anaplastic astrocytoma shows a large, heterogeneous mass (arrow heads) occupying much of the left cerebral hemisphere. (b) Axial diffusion-weighted image shows an area of restricted diffusion in this highly cellular tumor (arrow heads). (c) Axial apparent diffusion coefficient (ADC) map shows that the area of high signal on the diffusion-weighted image correlates with low signal (arrow heads) in the same area on the ADC map
One of the most active areas of research is in functional MRI (fMRI). fMRI relies on a blood oxygen-level-dependent (BOLD) technique to measure regional cerebral blood flow in response to a defined stimulus (Fig. 58.9) [48]. Several studies have shown that fMRI is an accurate method of identifying areas of neurologic function prior to resection of a brain tumor [49, 50]. Functional mapping using fMRI allows patients and their families to make informed decisions when there is a choice between maximal tumor resection and optimal functional outcome.
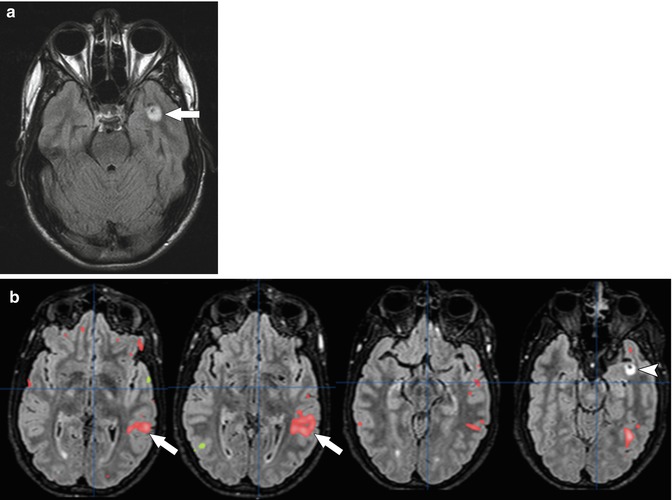
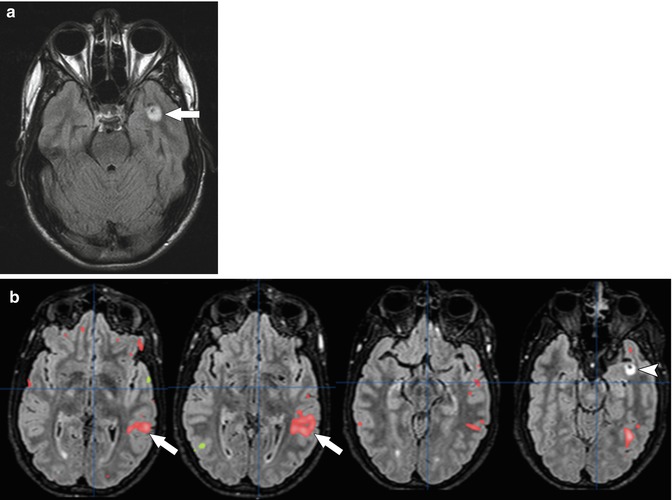
Fig. 58.9
(a) Axial FLAIR MR image in a 16-year-old with a left temporal lesion shows a focal area of signal abnormality (arrow) in the left temporal lobe. (b) Selected post-processed axial images from fMRI show increased signal in the left parietotemporal lobe (arrows) during a verb generation task. This suggests left-lateralized language and confirms that surgical resection of the lesion (arrow head) will have limited impact on language in this patient
Another type of functional MRI is tractography with diffusion tensor imaging (DTI) (Fig. 58.10). DTI is a technique that measures the directional diffusivity of water molecules. Because there is substantial directionality to the movement of water molecules in white matter tracts, DTI allows identification and mapping of these tracts [51]. Preoperative and intraoperative tractography has been used successfully in a number of pediatric brain tumors to determine the location of the white matter tracts and the effect the tumor is having on these tracts [51–53]. Knowledge of the course of these tracts also allows the surgeon to plan their approach via the most accessible route, while, at the same time minimizing damage to the brain [51].
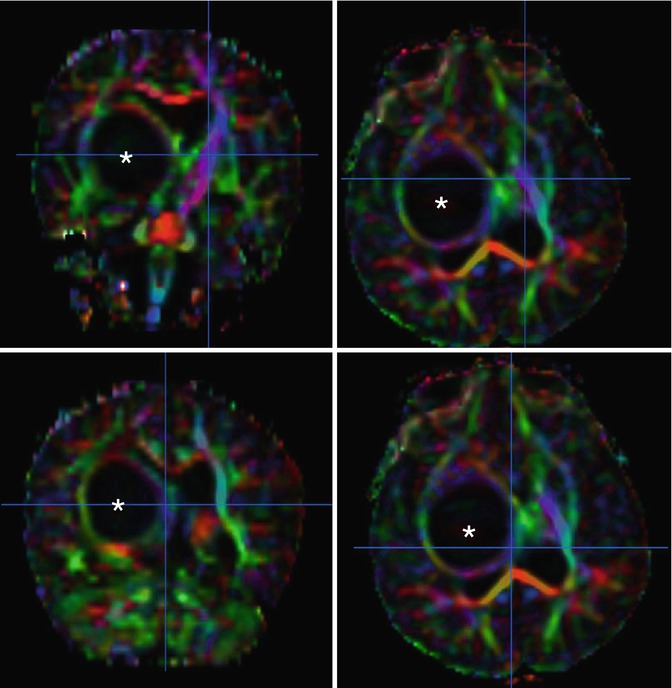
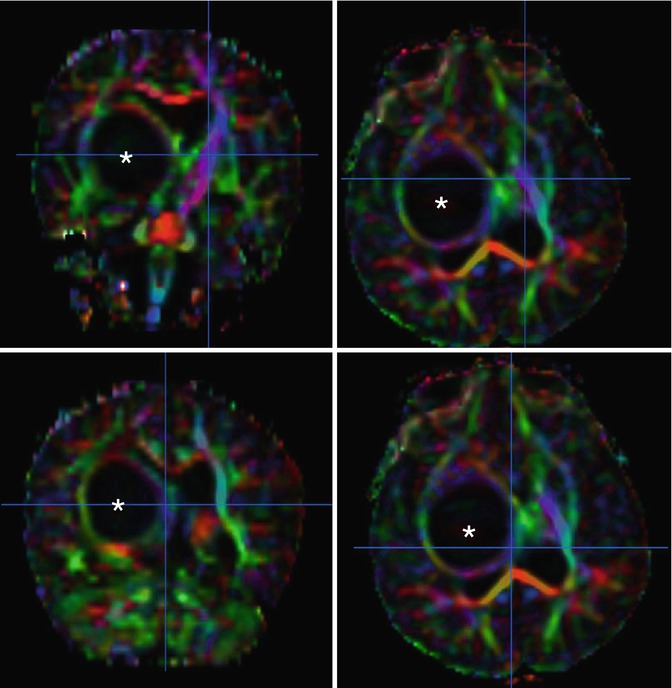
Fig. 58.10
Processed axial diffusion tensor images (DTI) in a 16-month-old with a right thalamic pilomyxoid astrocytoma show the tumor (asterisk) distorting right-sided white matter tracts
MR spectroscopy is a technique that can detect metabolic abnormalities in brain tissue (Fig. 58.11) [54]. It has been used to help differentiate tumor from other entities both at diagnosis and in follow-up after therapy. The major metabolites assessed in tumor imaging are N-acetylaspartate (NAA), a marker of neuronal integrity; choline, a marker of cell membrane and myelin turnover; lactate, a marker of anaerobic metabolism; and lipids, a marker of cell membrane destruction. In most brain tumors, there is a decrease in NAA and an increase in choline. Lactate and lipid peaks are present in areas of necrosis [37].
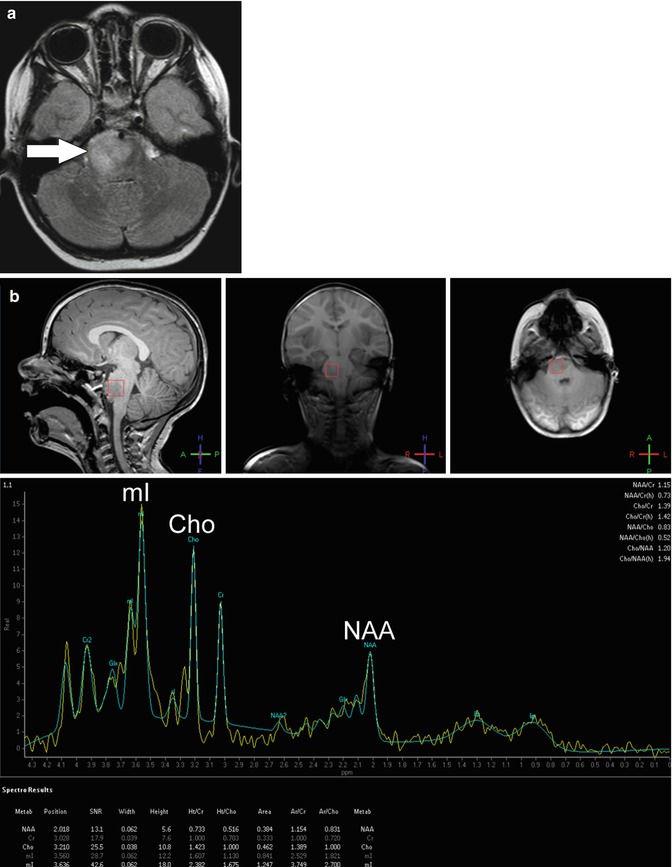
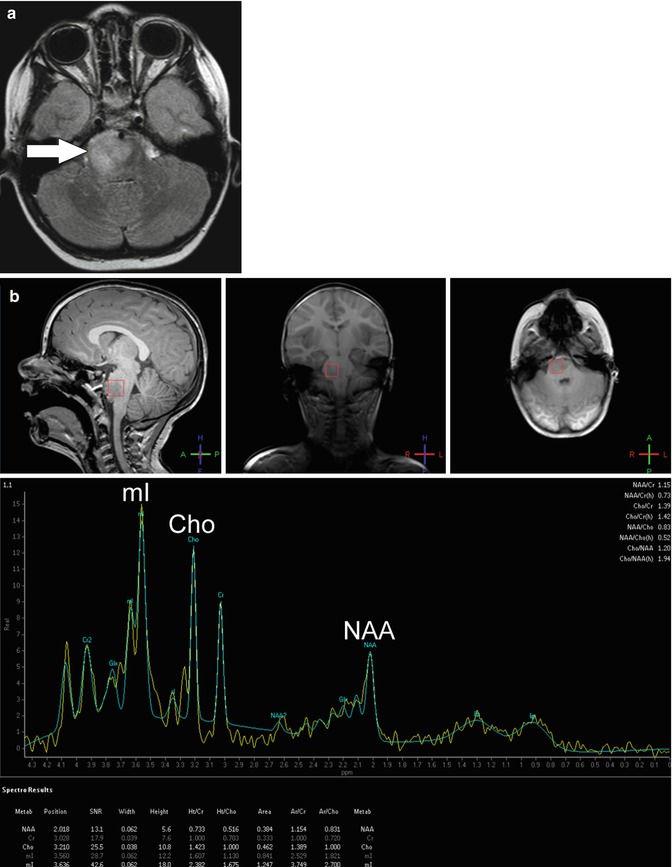
Fig. 58.11
(a) Axial FLAIR image in a 3-year-old with diffuse intrinsic pontine glioma shows asymmetric enlargement and increased signal of the right pons (arrow). (b) Screen capture from MR spectroscopy centered at the area of signal abnormality reveals a decreased N-acetylaspartate (NAA) peak and increased choline (Cho) and myoinositol (mI) peaks compatible with tumor
MR perfusion is a noninvasive method of assessing tissue hemodynamics (Fig. 58.12). Parameters measured include cerebral blood volume, the volume of blood in a defined region of brain per 100 g of brain tissue; regional cerebral blood flow, the volume of blood passing through a defined region over a specified time period; and mean transit time, the average time it takes for blood to pass through a defined region of brain tissue [37]. MR assessment of tumor perfusion has been used to evaluate angioneogenesis associated with malignancy. Cerebral blood volume is elevated in brain tumors as compared to normal brain and is somewhat correlated with tumor grade [37]. In general, low-grade gliomas have lower relative cerebral blood volume than high-grade gliomas [37]. Regional cerebral blood flow and the mean transit time also correlate with tumor angiogenesis, mitotic index, biology, grading, and response to therapy [37].
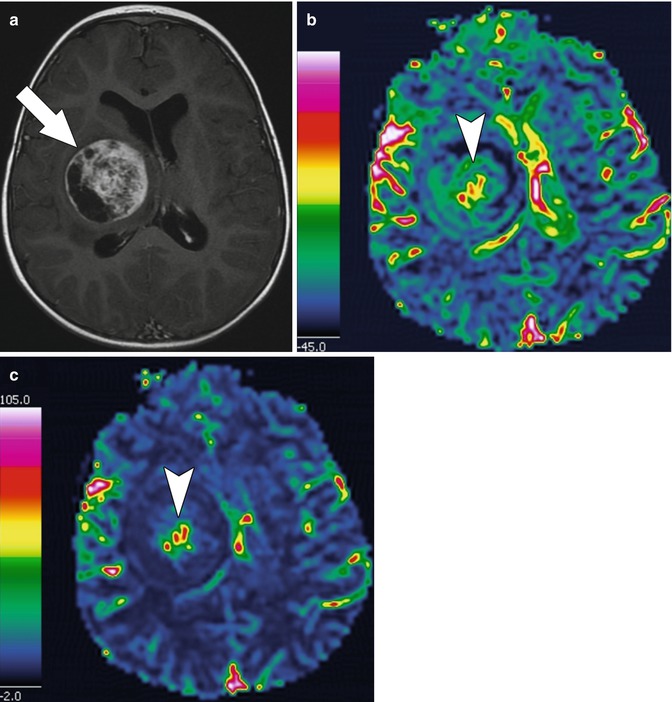
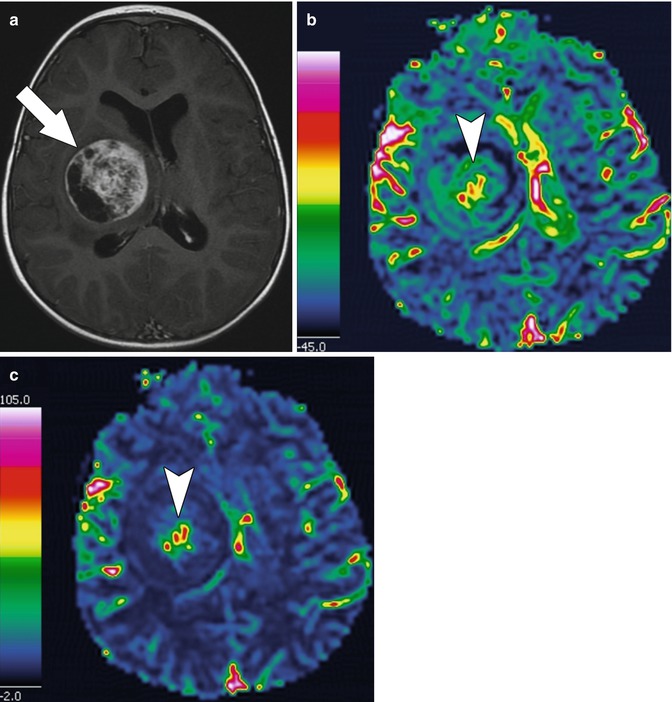
Fig. 58.12
(a) Axial T1-weighted post-contrast MR image in a 16-month-old with a right thalamic pilomyxoid astrocytoma (same patient as in Fig. 58.9) shows a large, heterogeneously enhancing mass (arrow) in the right thalamus that exerts mass effect. (b) Post-processed axial MR perfusion image of cerebral blood volume shows that the blood volume is increased centrally within the tumor (arrow head) compared to the surrounding normal white matter. (c) Post-processed axial MR perfusion image of regional cerebral blood flow shows increased blood flow (arrow head) centrally within the tumor reflecting angiogenesis
58.6 Neuroblastoma
Neuroblastoma is the most common extracranial solid tumor of childhood, accounting for 7–10 % of malignancies [55]. It is an embryonal tumor derived from neural crest cells. The tumors most commonly originate from the adrenal glands but can arise anywhere along the paraspinal sympathetic chain. Children typically present before 2 years of age with a painless abdominal mass. Symptoms can include malaise, irritability, weight loss, and/or neurologic symptoms such as opsoclonus-myoclonus.
Metastases are present at diagnosis in nearly 50 % of patients, with bone marrow, cortical bone, and liver being the most common sites of tumor spread [55, 56]. The 5-year event-free survival depends on a number of factors, including the patient’s age at diagnosis, molecular markers, and stage of disease [56]. The overall survival rate of patients who present with metastatic disease is less than 40 %. Survival decreases to less than 10 % for patients who fail to achieve a complete remission with induction therapy and for patients who have disease relapse [57]. Correct staging at the time of diagnosis is important to help oncologists initiate appropriate therapy and provide the family with a long-term prognosis.
Functional imaging plays an important role in the staging, follow-up, and therapy of patients with neuroblastoma. 123I meta-iodobenzylguanidine (MIBG) is the most common radiopharmaceutical used to diagnose and stage patients. It is an analog of norepinephrine that is normally taken up in the heart, lungs, salivary glands, liver, spleen, and adrenal glands with no normal uptake in the bones (Fig. 58.13) [58]. The primary route of excretion is through the urine leading to activity in the kidneys and bladder. Activity is also normally seen in large and small bowel.
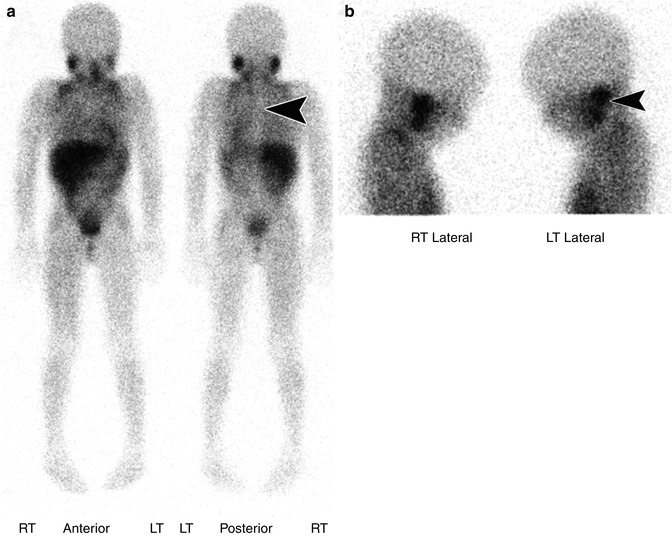
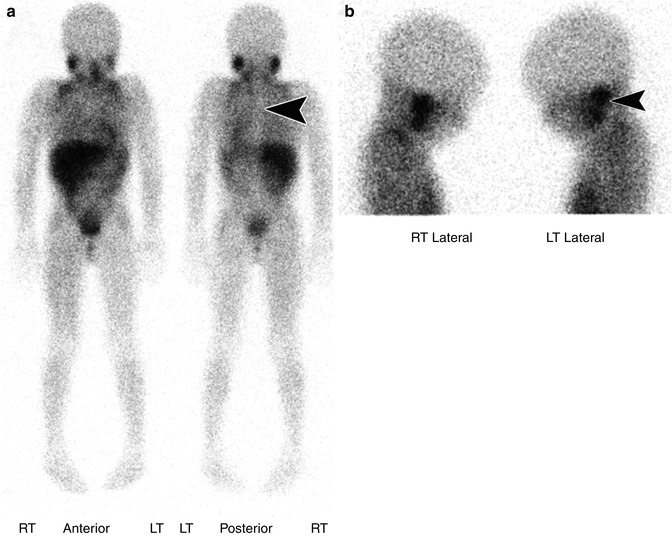
Fig. 58.13
(a) Anterior and posterior whole body images from an 123I MIBG scan in a 6-year-old with history of neuroblastoma status post-chemotherapy show normal physiologic distribution of MIBG with uptake in the salivary glands, heart, liver, spleen, bowel, and bladder. Symmetric uptake is also seen in metabolically active (brown) supraclavicular fat – a common finding. Note there is no tracer accumulation in the bone. On the posterior projection, the spine is seen as a vertical area of photopenia at the midline (arrow head). (b) Bilateral lateral views of the skull from an 123I MIBG scan show the physiologic uptake in the adjacent parotid and submandibular glands (arrow head)
There are several important elements to optimize imaging with 123I MIBG. Sympathomimetic and other medications that interfere with the uptake of MIBG must be discontinued in advance of imaging. Patients should receive oral potassium iodide either prior to or at the time of MIBG administration to limit thyroid uptake of 123I. Images should be obtained using a medium-energy collimator at 24 h after 123I MIBG administration with supplemental images the day of administration and at 48 h as needed. Typical images include whole body images and bilateral lateral views of the skull.
The staging of neuroblastoma is based on the anatomic extent of disease. MIBG is sensitive to the presence of disease with uptake in 90–92 % of tumors [56, 59]. MIBG is also very specific with very few false positives. For this reason, any uptake in nonphysiologic tissue is indicative of primary or metastatic neuroblastoma [58]. When there is a question of the anatomic location of uptake, SPECT/CT images can be performed. SPECT/CT with MIBG has been shown to improve diagnostic certainty by allowing better delineation of tumor from physiologic activity (Fig. 58.14) [60].
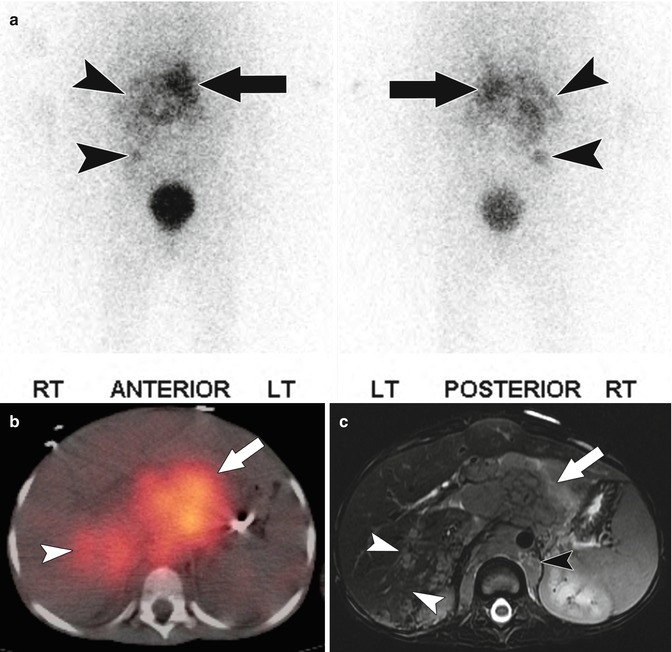
Get Clinical Tree app for offline access
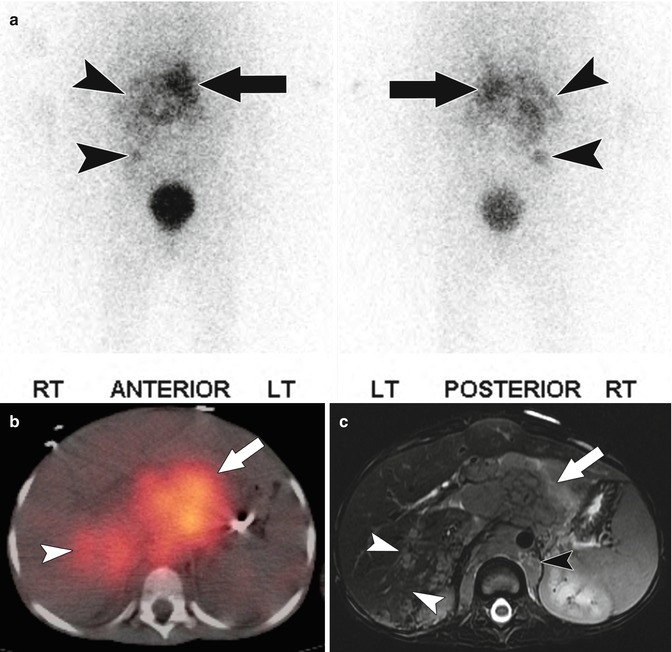
Fig. 58.14
(a) Anterior and posterior projections from an 123I MIBG scan in a 6-year-old with stage IV neuroblastoma show abnormal uptake in the upper abdomen at midline within the patient’s dominant mass (arrow) as well as other foci of abnormal MIBG uptake, in the right hemiabdomen (arrow heads). (b) Fused axial SPECT/CT 123I MIBG image of the upper abdomen shows uptake of MIBG within the dominant mass anterior to the spine (arrow) as well as abnormal uptake within the posterior segment of the right hepatic lobe (arrow head). (c) Axial T2-weighted image of the abdomen shows the large, infiltrative retroperitoneal mass (arrow) which extends posterior to the diaphragmatic crura (black arrow head
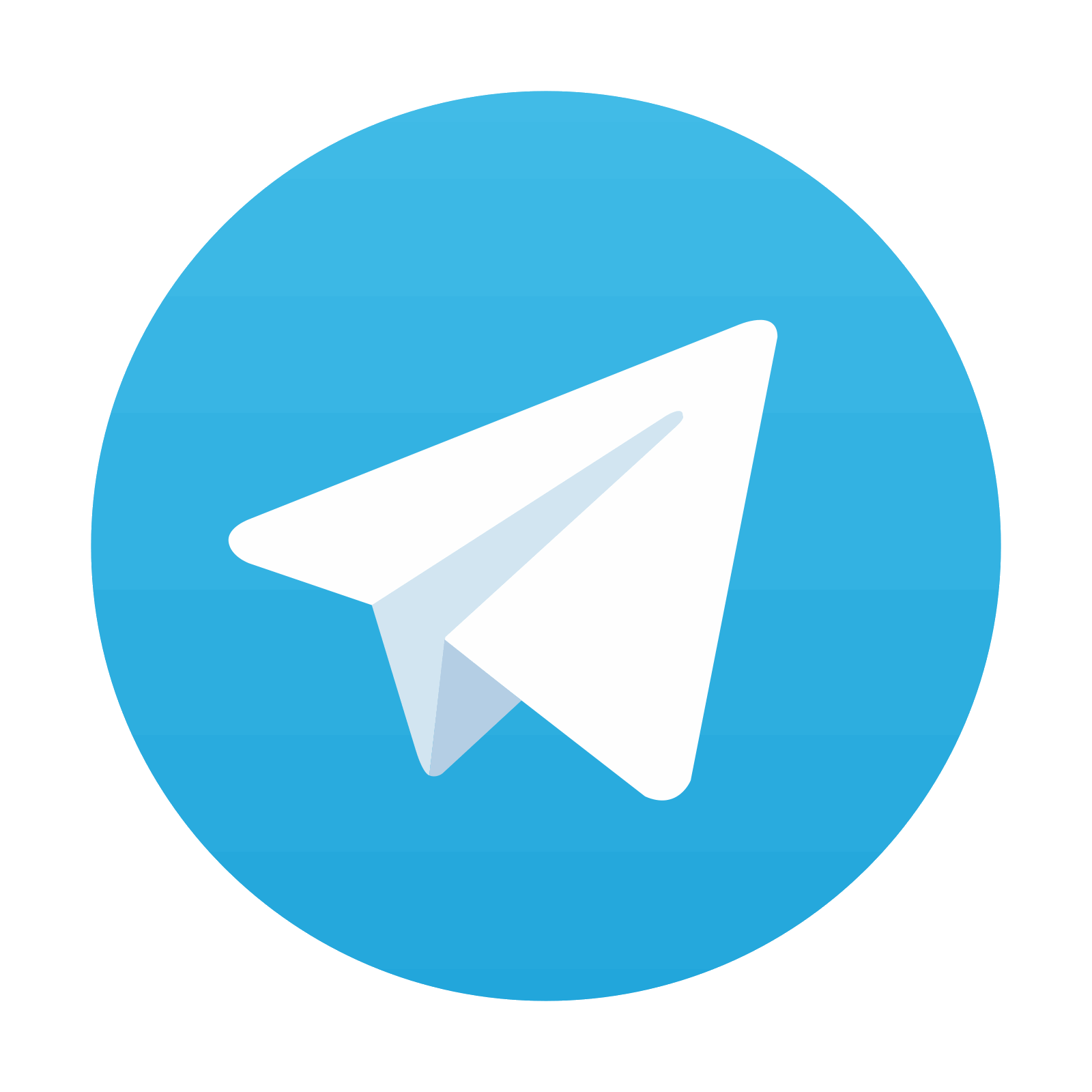
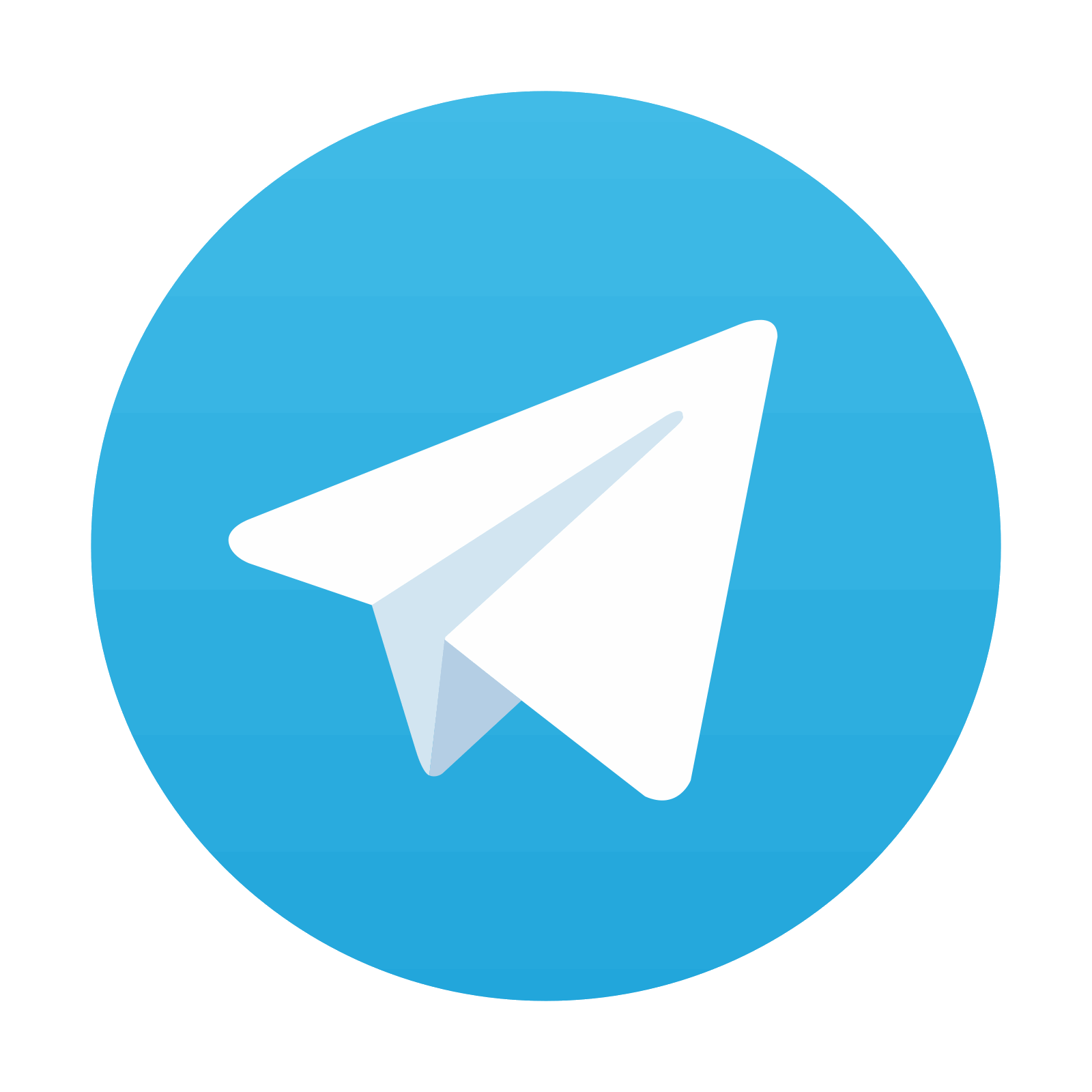
Stay updated, free articles. Join our Telegram channel
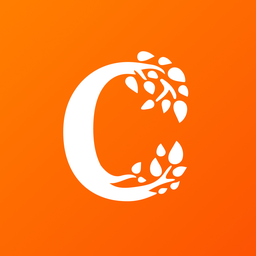
Full access? Get Clinical Tree
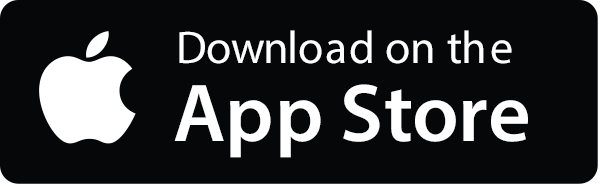
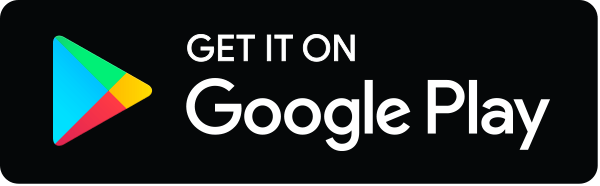
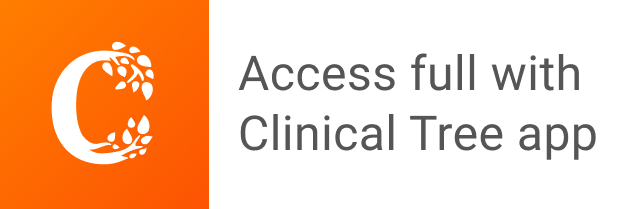