Fig. 12.1
Cellular and architectural microscopic morphology of the normal aortic valve (Adapted from Rajamannan et al. [23])
12.4.3 μOCT Imaging of Aortic Valves
Investigation of heart valve calcification has lagged that of coronary artery calcification. However, based on the concept that calcific aortic valve disease may represent one direction of the end stages of atherosclerosis, as coronary artery disease does, our laboratory has explored the use of μOCT in visualizing the pathological features associated with the early initiation and progression of calcific aortic valve disease [29].
We imaged human and murine aortic valve leaflets ex vivo [2011P000970]. Human valves were harvested from autopsy heart explants and surgical fragments obtained from aortic valve replacement surgeries. Each human aortic valve was dissected from the root of an aorta and placed in a sample chamber. The aortic valve leaf was partially submerged in phosphate-buffered saline to prevent tissue dehydration during image acquisition. The valve tissue was further sectioned into 2 mm stripes to match the μOCT system image acquisition range and to enable localization of the scanned area for histopathological validation. We chose regions of interest after gross visual inspection of the tissue and applied conventional OCT examination at room temperature. After imaging, we placed ink dots at the beginning of the scan volume to be able to identify the initial scan plane. Acquired images were processed with MATLAB and Image J [41]. We used H&E, Masson’s trichrome, and von Kossa stains for the histological study. Each μOCT image was correlated with histology.
We also imaged aortic valves from wild-type and ApoE knockout mice ex vivo to identify pathological features of early aortic valve calcification. Mice received a high-cholesterol diet for 20 weeks. The aortic root was dissected after the mice were euthanized. The valves were placed in the sample chamber with care not to destroy the tissue and were immersed in PBS during imaging at room temperature. Because the murine valves were too small to place registration ink dots, we matched histology by comparing each slide to two-dimensional recuts of the 3D μOCT dataset. Three-dimensional renderings of the μOCT images also provided information pertaining to gross valve morphology (Fig. 12.2). Histology was stained with von Kossa for detecting calcium deposition and alkaline phosphatase for visualizing early osteogenesis.
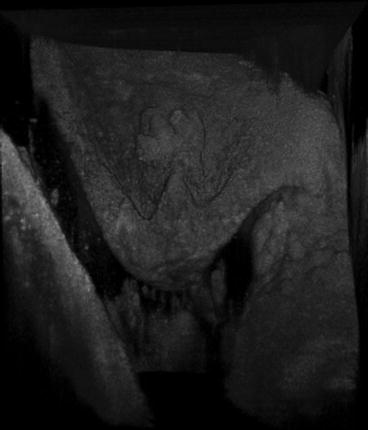
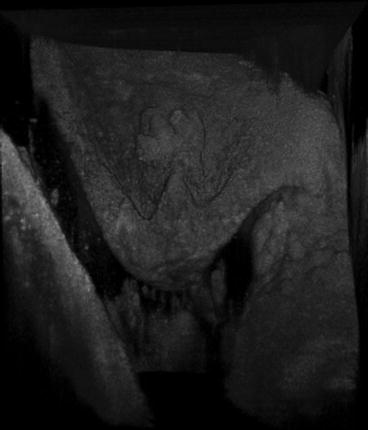
Fig. 12.2
3D reconstruction of a murine aortic valve cusp
12.4.4 Extracellular Matrix
The role of the ECM is to maintain valve homeostasis against mechanical forces of the aortic valve. Previous in vitro work suggested disarrayed or decreased collagen layer contributed to increased proliferation of valvular interstitial cells (VICs), apoptosis, and valve calcification [30]. The unique aortic valve structure contributes to valve pliability, enabling 〜100,000 open/close cycles a day and withstanding lifelong exposure to high aortic pressure. Normal valves exhibit organized ECM layers and compartmentalization of VICs, while diseased valves are marked by increased thickness, ECM disorganization, and VIC disarray [26, 27].
In our μOCT datasets, we were also able to identify similar disorganized structure in the diseased human valve. Correlated H&E histology demonstrated thickened fibrosa layers and decreased VICs (Fig. 12.3). Disorganized collagen fibrils are visible with Masson’s trichrome stain, which labels collagen as blue [23] (Fig. 12.4). Other μOCT images show a comparison between murine aortic valves of wild-type mice and ApoE-deficient mice given a high-fat diet. The organized ECM layers and compartmentalization of VICs can be seen in cross-sectional μOCT images of the mid-level cusps (right). A thickened, disorganized ECM is visible in the diseased valve (Fig. 12.5).
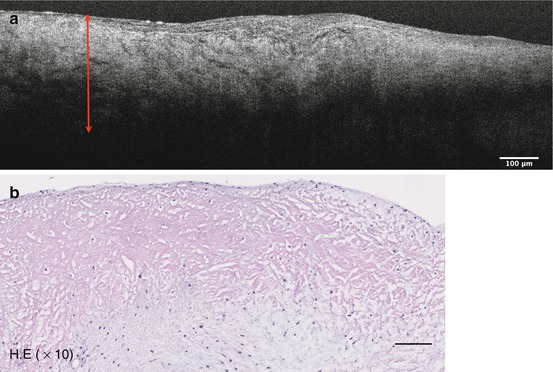
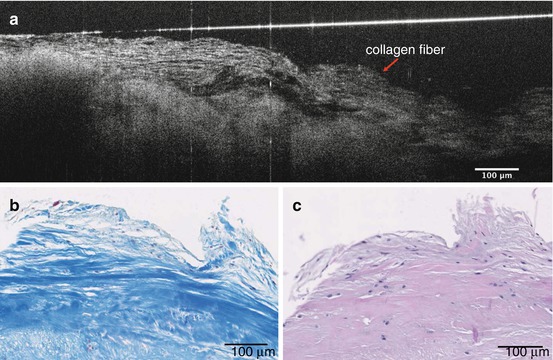
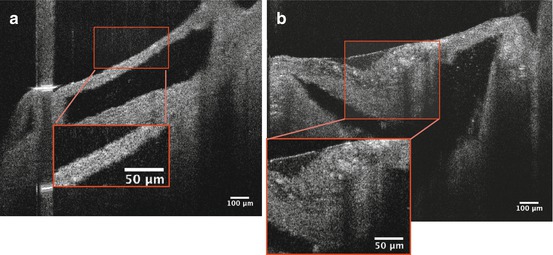
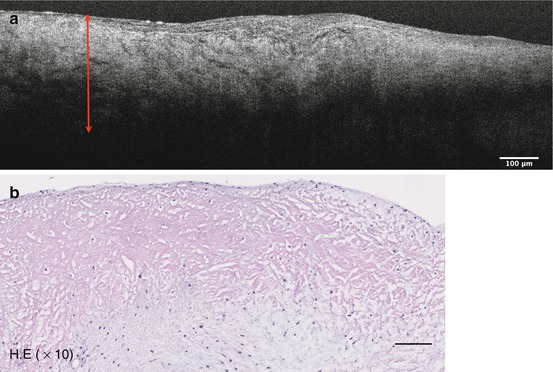
Fig. 12.3
μOCT image of the disorganized ECM (bidirectional arrow) of the diseased valve (a). Correlated histology shows thickened fibrosa layer and decreased VICs (b)
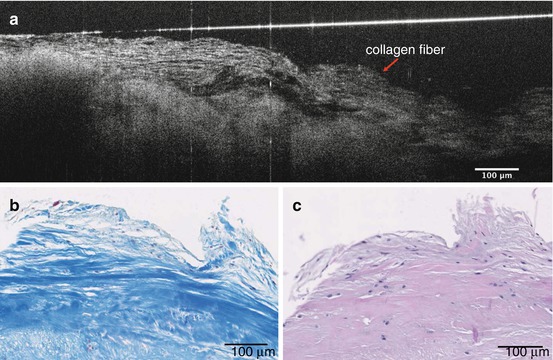
Fig. 12.4
μOCT image of disorganized collagen fibers (red arrow) (a). Correlated histology stained by Masson’s trichrome (b) and H&E (c)
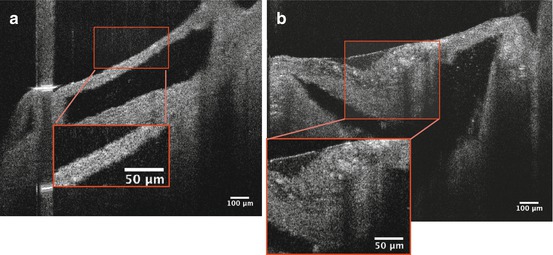
Fig. 12.5
Comparison of murine aortic valves between wild-type mice and ApoE-deficient mice. A normal valve has organized ECM layers and compartmentalized valvular interstitial cells (VICs), as shown in a wild-type leaflet (a, red box). Valve thickness is on the order of 50 μm and organized elastin fibers are clearly visible in the aortic root. A diseased valve exhibits thickened (>100 μm) and disorganized ECM (b, red box)
12.4.5 Cells
As in arterial atherosclerosis, various inflammatory cells such as activated macrophages, foam cells, and lymphocytes are involved in the mechanisms that underlie the development of CAVD. Once endothelial cells become inflamed, adhesion molecules not found in normal valves are expressed. Monocytes adhere to the adhesion molecules, migrate into the subendothelial space in the valve, and differentiate into activated macrophages [31]. Activated macrophages are thought to play a central role in the proteolytic action of early valve calcification by producing a variety of cytokines that cause ECM remodeling and consequently increasing valve stiffness and thickening. Aikawa et al. demonstrated the accumulation of macrophages in early aortic valve lesions using fluorescence reflectance imaging [32].
μOCT can readily visualize macrophages, seen in images as a distinct signal-rich region of oval shape. Correlated histology showed numerous macrophage accumulations in disorganized ECM stained by H&E (Fig. 12.6). In another representative image, a 30 μm large cell can be seen adherent to the endothelium, likely to be a monocyte based on size and shape (Fig. 12.7).
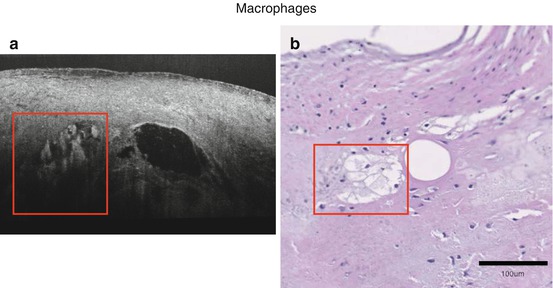
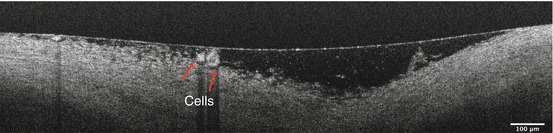
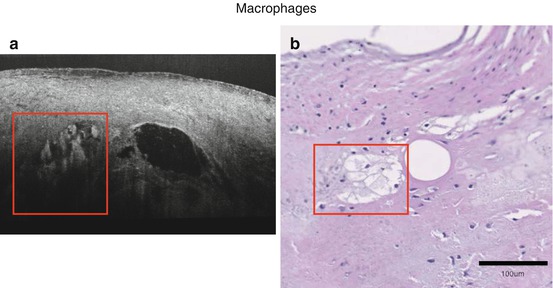
Fig. 12.6
Representative image of macrophages (a). Macrophages (red boxes) accumulate within the voids, supported by H&E histology (b)
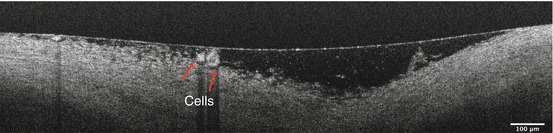
Fig. 12.7
Cells adherent to the endothelium (red arrows). 30 μm large cells are considered to be macrophages judging from the size and shape
12.4.6 Cholesterol
Cholesterol facilitates the vicious cycle of cardiovascular inflammation [29]. The initiation is saturated lipoprotein entry and accumulation in the arterial wall or subendothelium within the aortic valve, and oxidation, which invites the entry of macrophages. Accumulation of macrophage-derived foam cells leads to the formation of cholesterol crystals, finally resulting in foam cell apoptosis and extracellular lipid deposit [33]. Previous study suggested products of oxidized cholesterol may facilitate aortic valve calcification [34, 35]. Duewell et al. demonstrated cholesterol crystal appearance in early atherosclerotic lesions and that the NLRP3 inflammasome cytokine pathway is triggered by cholesterol crystals and results in acute inflammation [36]. On μOCT images, cholesterol crystals are seen as signal-rich needlelike structures. A single cholesterol crystal penetrating the cap of the necrotic core is resolvable by μOCT [16] (Fig. 12.8). The high resolution of μOCT appears to lower the threshold of detection for cholesterol crystals, which have been inconsistently visualized by previous lower resolution OCT technologies [9]. Similar to coronary artery atherosclerosis, lipid accumulation is found in early lesions of calcified valves and may accelerate valve calcification [29, 31, 37, 38]. Cholesterol crystals may be seen as fine linear structure adjacent to huge necrotic core in our μOCT images (Fig. 12.9). It is potentially useful to detect cholesterol deposition in an early stage of CAVD, which could enable the discrimination of high-risk patients that may undergo subsequent disease progression.
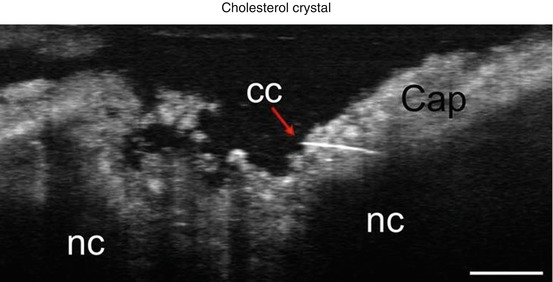
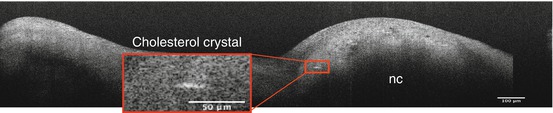
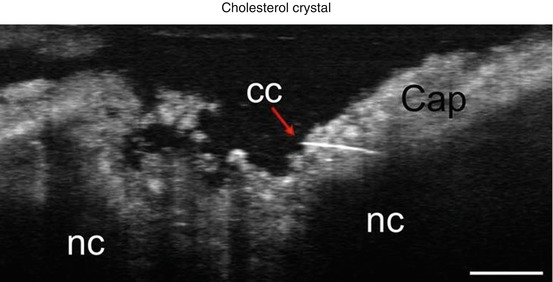
Fig. 12.8
Representative image of cholesterol crystal (red arrow), which penetrates into the cap of a necrotic core within a human coronary artery. Scale bar, 30 μm. CC cholesterol crystal, NC necrotic core (Reproduced from Linbo et al. [16])
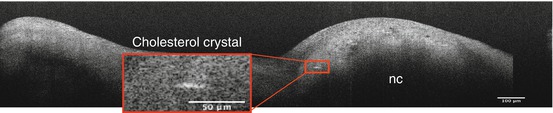
Fig. 12.9
A linear structure consistent with a cholesterol crystal identified in a human aortic valve
12.4.7 Microcalcification
Microcalcification is an outcome of the mineralization process in the aortic valve. Microcalcifications may enhance inflammation and contribute to more abundant deposition of calcium and increased valve stiffness [23, 39]. Vengrenyuk et al. hypothesized that microcalcification in a fibrous cap may cause stress concentration and contribute to rupture based on finite element analysis (FEM) and high-resolution imaging, including confocal microscopy and micro-computed tomography, which confirmed the existence of microcalcifications in autopsy samples ex vivo [40].
μOCT can identify microcalcifications as accumulations of small, punctate high signal densities adjacent to a large necrotic core (Fig. 12.10). Correlated histology shows microcalcifications stained by von Kossa and a large necrotic core (Fig. 12.10). Figure 12.11 shows an aortic valve of ApoE-deficient mice subject to a high-fat diet, imaged by μOCT. Microcalcification is visualized as distinct punctate densities in the thickened diseased valve, supported by correlated histology with von Kossa staining (Fig. 12.11).
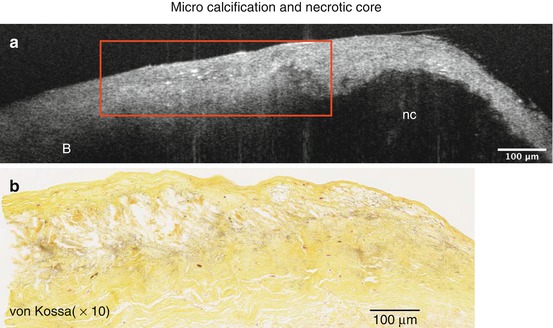
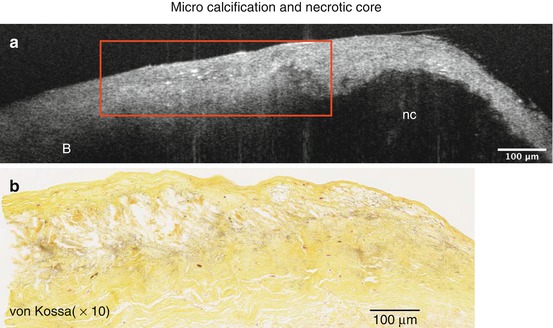
Fig. 12.10
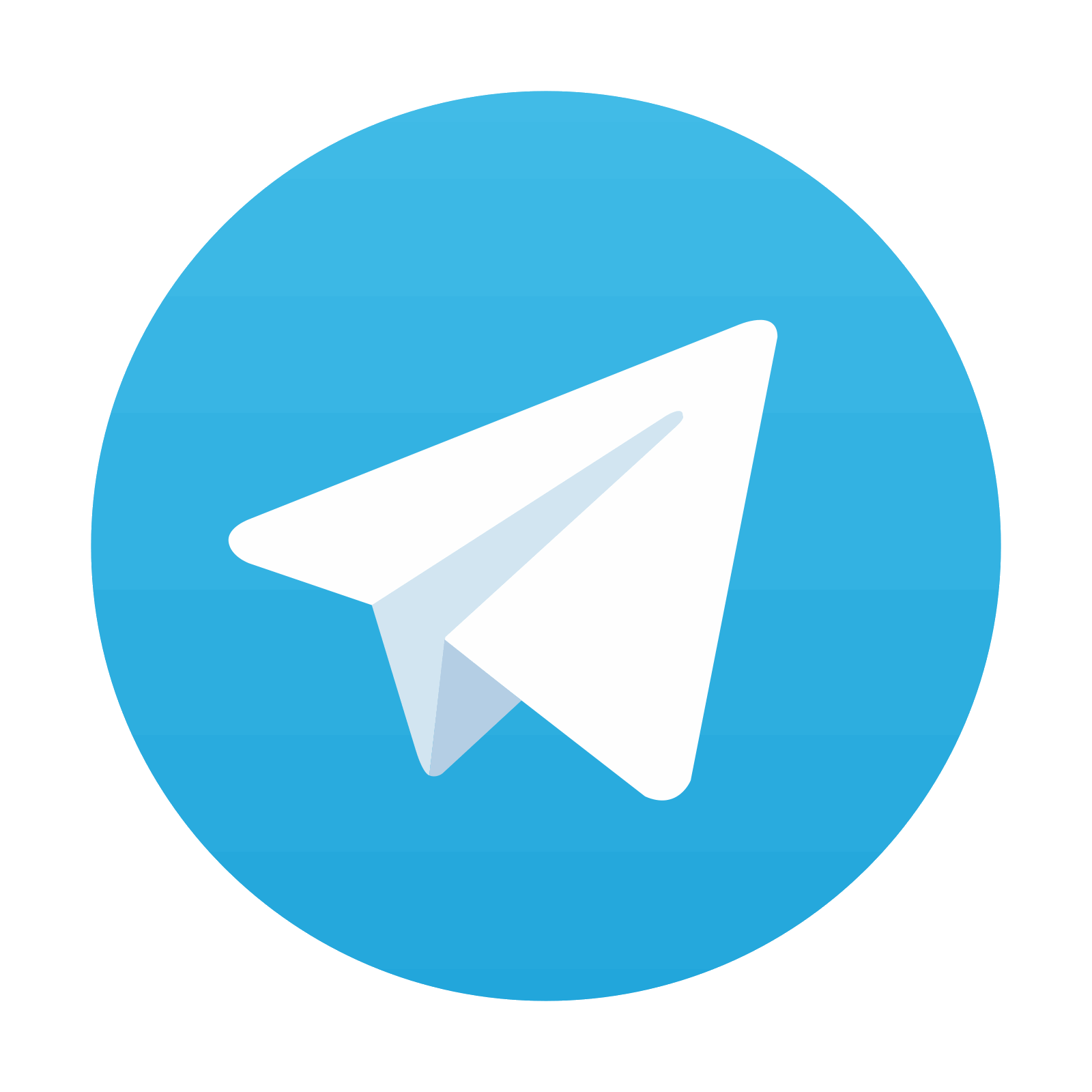
Microcalcification adjacent to necrotic core (a). Microcalcification (red box) appears as an accumulation of small, punctate high signal densities in μOCT images, supported by corresponding von Kossa stain (b)
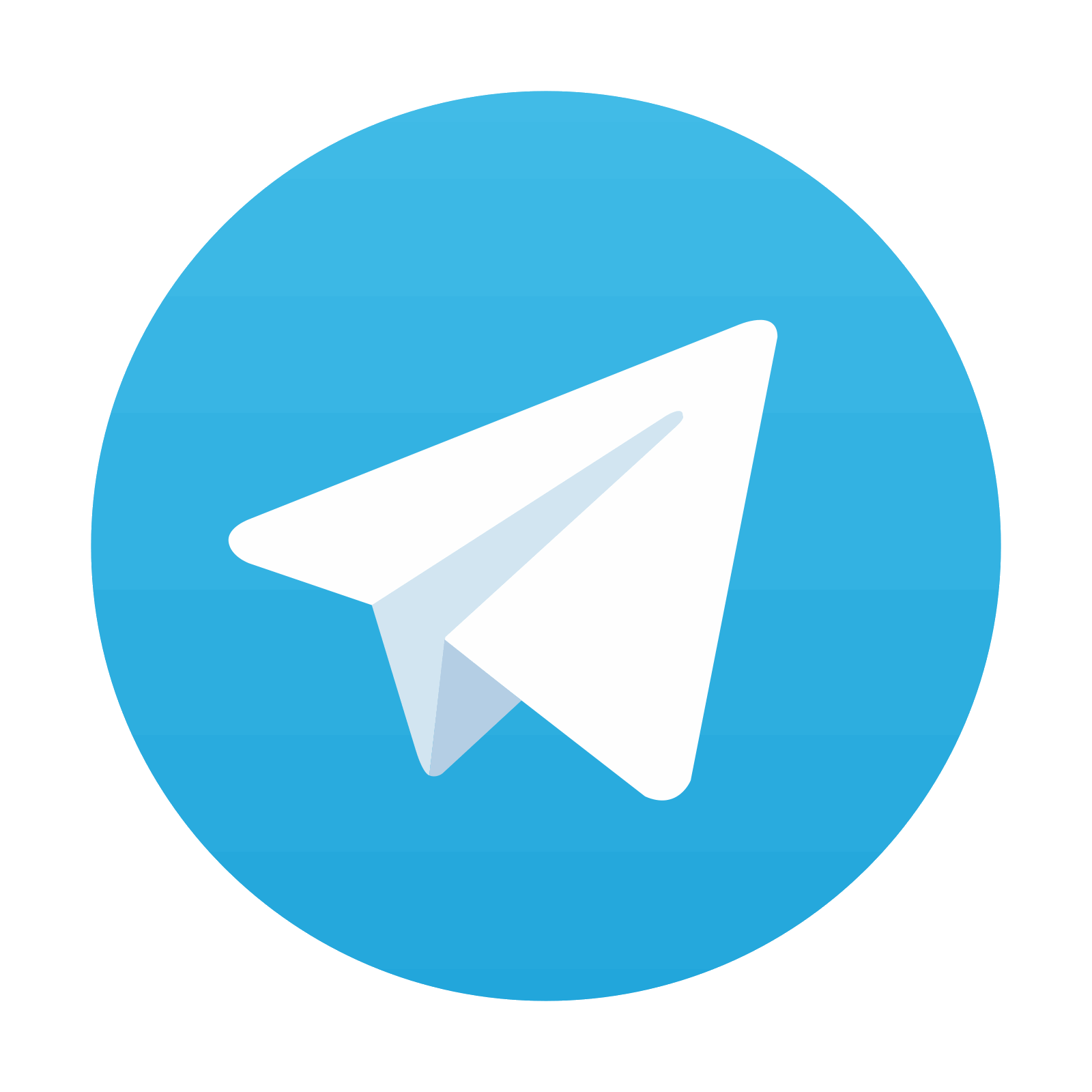
Stay updated, free articles. Join our Telegram channel
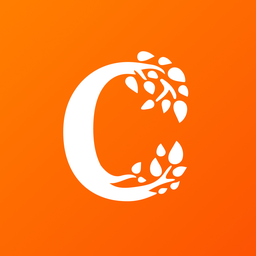
Full access? Get Clinical Tree
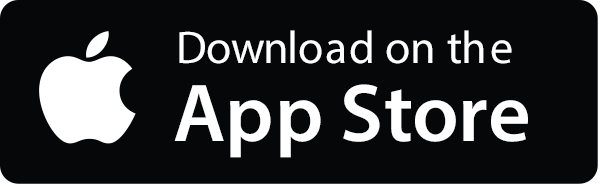
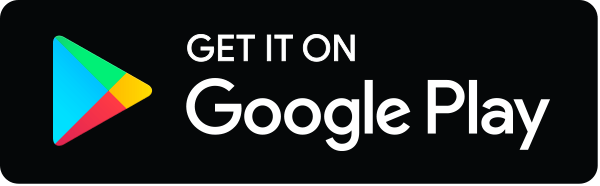
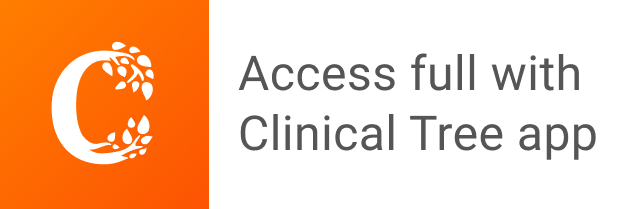