Fig. 1
The time course of liver symptoms in patients treated with radiation therapy (with permission from Rubin and Casarett 1968)
2 Anatomy and Histology
2.1 Anatomy
2.1.1 Gross Anatomy
The liver is the largest internal organ in the human body, representing slightly less than 3 % of body weight, weighing 1,200–1,500 g, but receiving 25–30 % of the cardiac output. It is located in the right upper abdominal quadrant within the peritoneal cavity.
During weeks two to three of development, the gastrointestinal tract (GIT) arises from the endoderm of the trilaminar embryo. The three distinct portions, an anterior foregut, a central midgut, and a posterior hindgut ultimately contribute different components of the GIT. The foregut includes hepatic cells and bile ductules, gall bladder and common bile duct, pancreatic acinar and island cells, and duodenum. The liver and biliary tree appear late in the third week or early in the fourth week as the hepatic diverticulum, an outgrowth of the ventral wall of the distal foregut. The hepatic diverticulum subsequently divides into a small ventral part, the future gall bladder, and a larger cranial part, the liver primordium; the latter portion grows and differentiates into the parenchyma of the liver (hepatocytes) and the lining of the biliary ducts. The hepatocytes arrange into a series of branching and anastomosing plates in the mesenchyme of the transverse septum. These plates subsequently intermingle with vitelline and umbilical veins to form hepatic sinusoids. Besides contributing to the sinusoids, the splanchnic mesenchyme in the transverse septum also forms the stroma, the fibrous and serous coverings (liver capsule), the falciform ligament, and the blood forming, or hematopoietic tissue (Kupffer cells), of the liver. The connective tissue and smooth muscle of the biliary tract also develop from this mesenchyme. By the sixth week, the liver performs hematopoiesis (the formation of blood cells) and represents 10 % of the total weight of the fetus by the ninth week.
The liver is divided into two lobes: the right and the left, separated by the middle hepatic vein, which defines the imaginary line, the so-called Cantlie’s line, from the middle of the gallbladder fossa and corresponding to the plane of the inferior vena cava (IVC), which runs posteriorly to the liver. The right and left lobes are each drained by a major vein (the right and left hepatic veins, respectively). The middle hepatic vein and these two veins drain into the IVC on the superior-posterior aspect of the liver. There is variation to the overall vascular drainage, and in some people, the left and middle hepatic veins become confluent prior to emptying into the IVC.
Using the anatomic location of the three hepatic veins, the liver has been divided into seven segments, based on the Couinaud classification system. Each lobe is divided into four segments, I through IV on the left and V through VIII on the right with segment I representing the caudate lobe (Couinaud 1992). Each segment has its own vascular inflow, vascular outflow, and biliary drainage (Fig. 2).
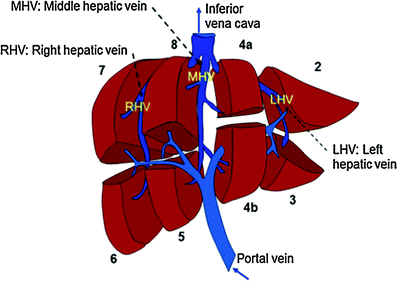
Fig. 2
The natural anatomy of portal vein flow and the right hepatic vein (RHV), middle hepatic vein (MHV), and left hepatic vein (LHV) drainage is utilized in the definition of hepatic segments; the caudate lobe (not shown here) represents segment 1
2.1.2 Vasculature
The liver receives dual blood supply from the portal vein (70 %) and hepatic artery (30 %). The hepatic artery is rich in oxygen, while the portal vein provides the liver with metabolite-rich but relatively poorly oxygenized blood. The portal vein takes its origin from the confluence of superior mesenteric vein and splenic vein, between the posterior surface of pancreatic neck and anterior surface of the IVC. The portal venous and hepatic arterial blood supplies parallel each other as they enter the portal triad and sequentially divide among the segments and subsegments of the liver.
The relationship of the liver to the portal vein is important in the understanding of portal hypertensive changes. Although the larger part of blood supply to hepatocytes is from the portal vein, the biliary system obtains all its supply from the hepatic artery. An injury to the hepatic artery therefore tends to have more severe effect on the biliary system than the hepatocytes. The portal vein is autoregulated, but the extent of arterial flow is partly dependent on the portal flow, such that situations reducing portal flow reflexively increase arterial flow. The mean pressure in the portal vein is 10 mm Hg compared to 90 mm Hg in the artery. However, the intrahepatic regulation ensures the arterial pressure does not extend into the sinusoids, which have a mean pressure of 5 mm Hg. Any pathologic process interfering with sinusoidal flow could have a far-reaching effect on this arterial pressure regulation and hepatic hemodynamics. This has relevance, as described in Sect. 3.3.2, in the pathogenesis of sinusoidal obstruction syndrome.
In summary, the liver receives dual arterial and venous blood supply, which flow through a system of sinusoids within the hepatic parenchyma in well-regulated pattern, to empty through small hepatic veins, that in turn drain into one of three major hepatic veins, and ultimately into the IVC. Details of the intrahepatic flow through the sinusoids are discussed in the next section—microanatomy.
2.2 Histology (Microanatomy)
The flow from the portal vein and hepatic artery enter the hepatic hilum soon after each divides into the right and left branches. Subsequent intrahepatic divisions create up to 1,000,000 interlobular branches of the portal vein and hepatic artery. The biliary system drains in the opposite direction but follows similar architecture, such that interlobular bile ducts (that drain bile canaliculi) progressively confluent to form the right and left hepatic ducts, which exit at the hilum, and fuse to become the common hepatic duct. The point where the cystic duct draining the gallbladder joins the common hepatic duct represents the beginning of the common bile duct, which eventually empties into the duodenum at the ampulla of Vater. Lymph flows in the same direction as bile. Thus, each interlobular portal tract consists of (at least) an arteriole, a venule, a bile duct, and lymphatic vessels enmeshed within a fibrous connective tissue, hence the term portal triad. Figure 3a shows the low magnification hepatic lobules and Fig. 3b shows the high magnification intralobular structure.
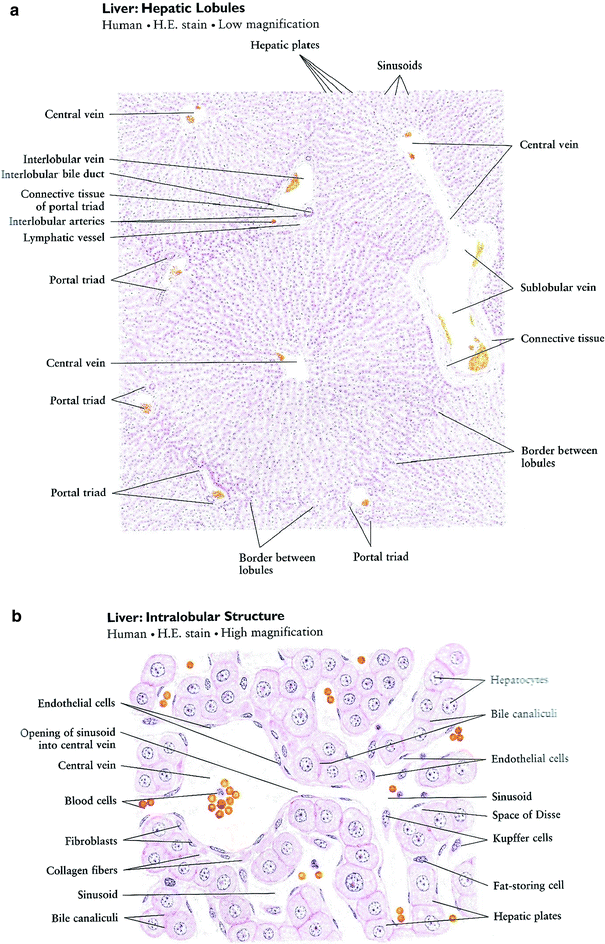
Fig. 3
Liver histology. a Low magnification. b High magnification (with permission from Zhang 1999)
Surrounding the connective tissue is a single layer of hepatocytes running concentric to the triad and termed the limiting plate. The next layers of hepatocytes are arranged perpendicular to this plate running from the portal tract to the central vein (strictly a venule from its size). Separating the hepatocyte cords from each other is a system of sinusoids, lined by hepatocyte-specific fenestrated endothelial cells with a different immunophenotype from endothelial cells elsewhere in the body (see below). They function to regulate the passage of material between the sinusoids and subendothelial space that is in direct contact with hepatocytes. This perisinusoidal space (space of Disse) houses hepatic stellate cells (Ito cells) and native liver macrophages (the Kupffer cells). The stellate cells are storage site for vitamin A, and can become activated to assume myofibroblastic phenotype and function, playing significant role in fibrosis formation in most chronic liver injuries. After traversing the sinusoids, the vascular outflow proceeds to the central veins, the sublobular veins, and then to the suprahepatic vein that ultimately drains into the IVC.
Endothelial cells, with two types of phenotypes, line the entire vascular system of the liver. The endothelial cells lining the portal veins, hepatic artery, hepatic venules, and hepatic veins exhibit systemic phenotype in that they are continuous and express among others, CD34 and factor VIII-related antigens, as would be expected elsewhere in the body. In contrast, the endothelial cells lining the sinusoids are fenestrated and do not express these antigens. In pathologic states such as neoplasms and cirrhosis, sinusoidal endothelium can revert to systemic phenotype (Couvelard et al. 1996).
3 Biology, Physiology, and Pathophysiology
3.1 Physiology (Functional Unit)
Traditionally, two structural systems have been considered as representing the functional unit of the liver, i.e., the smallest unit that represents the complete structure, function, and hemodynamics of the entire system: the acinar and the lobular systems. The lobule has a hepatic venule at its center with 3–6 vascular inflow portal triads arranged at the periphery of a polygonal unit (Fig. 3). Blood flows from the portal triad toward the central vein, and the oxygen concentration accordingly decreases toward the perivenous sinusoids. This does not truly represent the entire complex, as blood flows in the opposite direction to the lobular concept. For this reason, Rappaport defined the acinar concept as being more representative of the true function and hemodynamics of the liver (Rappaport 1976). The hepatic acinus includes the portal tract at its center, where blood and nutrients enter the unit and the central vein at the periphery or distal end, where the liver is drained. The hepatocytes closest to the portal tracts are functionally and metabolically different from the ones further away, around the hepatic venule (Jungermann 1988). Among these differences is their relative susceptibility to various forms of injury; for example the distal (peri-venular) hepatocytes are more susceptible to hypoxic/ischemic injuries than the more proximal hepatocytes. The proximal or periportal hepatocytes, also known as the zone 1 hepatocytes, are better oxygenized, but more susceptible to injury from some toxins that enter the liver via portal flow and are more concentrated at this zone. The distal hepatocytes in the unit are termed zone 3 hepatocytes, and those between zones 1 and 3, termed zone 2. Zones 1, 2, and 3 therefore correspond, respectively, to periportal, mid-zonal, and centrilobular (or perivenular) divisions of the lobular unit, as shown in Fig. 4. For most practical and descriptive purposes, the acinar unit is often utilized to describe the functional unit of the liver.
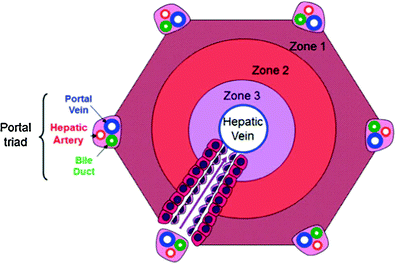
Fig. 4
Illustration of normal hepatic acinus illustration showing zones 1–3 as they relate to the portal tracts and hepatic vein. The arrow depicts direction of blood flow through the sinusoids
Within the liver, there are many functional subunits, with substantial redundancy.
3.2 Pathophysiology
3.2.1 Tissue, Cellular Mechanisms
The numerous redundant functional subunits within the liver allows the liver to endure substantial injury prior to development of any clinical sequelae. In noncirrhotics, surgical resection of a majority of the liver that leaves only a 20–25 % liver remnant has been shown to be well tolerated. Because of this redundancy, partial liver irradiation to high doses is possible using conformal radiation therapy if adequate normal liver parenchyma can be spared.
Due to the surplus of functional subunits in one liver, the architecture of the liver can be considered predominantly arranged “in parallel”. Seriality in vascular and biliary function exists, as the vascular structures and the biliary system have many bifurcations throughout the liver that ultimately drain to main vessels or the common bile duct. Injury to the main vessels or common bile duct will have more adverse clinical consequence that injury to a distal branch. Furthermore, large vascular or biliary injury may alter the function of the liver associated with these structures. Thus the liver function itself may be considered mainly “in parallel”, with potential for some “serial” function.
Regarding classic RILD, the underlying pathophysiology is poorly understood. However, injury to endothelial cells rather than hepatocytes has been postulated to be initiating, due partially to the timing of pathological changes observed in classic RILD (described in Sect. 3.2).
3.2.2 Morphological Appearance of RILD
The morphological appearance of classic RILD in humans is one of VOD, predominantly of the central and sublobular hepatic veins, with sparing of the periportal areas, larger hepatic veins, and hepatic arterial vasculature (Fajardo and Colby 1980; Reed and Cox 1966; Ogata et al. 1963) (Fig. 5c). While the lesion may affect anywhere from a portion of a lobule to the entire liver, it occurs strictly within the irradiated region and is sharply demarcated from adjacent unaffected areas of liver (Fig. 5a, b). Grossly, the involved liver appears markedly congested, and may contain areas of necrosis and parenchymal collapse in the center of larger involved regions.
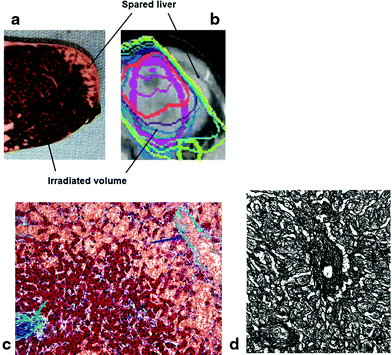
Fig. 5
a Gross photograph showing acute sinusoidal obstruction in resected left liver lobe following previous radiation therapy to a neoplasm (neoplasm not shown here), delivered 3 months previously. The sharp delineation of the change in the gross specimen is consistent with the radiation dose falloff, rather than anatomic regions. b Radiation plan: 30 Gy in 6 fractions (yellow), with sparing of the lateral left lobe. c Microscopy of acute sinusoidal obstruction in resected left liver lobe following radiation therapy; hepatic vein—blue arrow; portal tract—green arrow. The sinusoids and later the hepatic vein become prominent due to severe congestion (Trichrome stain, original magnification: 100x). (PDF Fig. 4). d Late fibrosis: typical centrilobular areas in moderately severe radiation-induced liver disease (RILD), fibrillary material (collagen) (with permission from Fajardo and Colby 1980 14)
In their classic description that established the features of RILD, Reed and Cox described in detail the progression of morphological changes that occur over the course of RILD (Reed and Cox 1966). In the early subacute period, typically the first month after RT, affected liver portions are markedly hyperemic, but display few venous lesions and only minimal evidence of outflow obstruction and hepatic cell loss. During the ensuing months, which correlate with the clinical presentation of RILD, veno-occlusive lesions become frequent and prominent, with severe vascular congestion and atrophy of centrilobular liver plates. The pathological hallmark of RILD, veno-occlusive lesions, are characterized by complete obliteration of central vein lumina by erythrocytes trapped in a dense network of reticulin and collagen fibers that crisscross the lumen of the central veins, sublobular veins, and centrilobular sinusoids. Fibrin appears to be deposited peripherally along the intimal walls of these vessels, rather than in center of the lumens as are collagen and reticulin. Collagen is present not only in the centrilobular veins, but appears to proliferate along the hepatic sinusoids and produce mild congestion in periportal areas as well. Centrilobular hepatocytes (zone 3) are largely absent, presumably due to hypoxic cell death secondary to vascular congestion. After approximately 4 months, vascular congestion resolves as the liver begins to gradually heal. Although asymptomatic, the hepatic architecture remains largely distorted, with persistent fibrosis of central veins, fibrous tracts bridging portal and central veins, and unrepaired lobular collapse prominent throughout the affected region (Fig. 5d).
A characteristic feature of RILD, which is not yet well studied in the other forms of VOD, is the deposition of fibrin strands with entrapment of platelets within the central veins as demonstrated by electron microscopy. The elevation of alkaline phosphatase more than the transaminases in classic RILD suggests that bile ducts or hepatic canaliculi may also be injured in irradiated livers.
Of note, thrombosis of larger hepatic veins, such as seen in Budd-Chiari syndrome, has been uncommonly seen following liver irradiation (Rahmouni et al. 1992). However, radiation-induced thrombosis is typically not associated with centrilobular coagulative necrosis and clinically does not usually induce jaundice, which is often seen in patients with Budd-Chiari syndrome.
3.2.3 Animal Models
The pathogenesis of RILD is difficult to investigate because of the lack of a suitable animal model. Rat (DeLeve et al. 2002), mice (Tabbara et al. 2002), dog (Reiss et al. 2002; Bearman 2001), and rhesus monkey (Shulman et al. 1980) models studied to date do not develop a similar pathology or clinical syndrome to that seen in humans (the VOD of classic RILD). However, the murine and rodent microvascular endothelial cells are sensitive to radiation injury (Shulman et al. 1987). Furthermore, perivenous atrophy of hepatocytes has been noted in rodent models of RILD, despite a lack of central vein occlusion by platelet thrombi (Shulman et al. 1994).
Geraci and colleagues first reported that rats receiving whole liver irradiation in excess of 25 Gy developed liver dysfunction after a latent period of 35–40 days (Geraci et al. 1991). In their study, hepatocellular injury was evident by elevations in AST, ALT, alkaline phosphatase, and 131I rose bengal retention, an indicator of hepatobiliary clearance. Morphologically, these rats appeared to develop a pathology that somewhat resembled the VOD described in humans, with venous outflow obstruction by collagen deposition, sinusoidal vascular congestion, interstitial fibrosis, and focal necrosis of centrilobular hepatocyte (Geraci et al. 1991). However, no studies to date of hepatic irradiation in rodents by other investigators have successfully reproduced these pathological findings. This may be due to the fact that unless the entire liver is irradiated, even a small spared portion can rapidly regenerate and prevent the clinical syndrome of RILD (Geraci et al. 1991). Thus, despite the promise of Geraci’s reports, a reproducible rodent model that mirrors human RILD remains elusive.
3.2.4 Viral Hepatitis
Another mechanism thought to contribute to the pathogenesis of nonclassic RILD is the subclinical or ongoing cirrhosis of the liver associated with hepatitis B virus (HBV). Most patients undergoing irradiation of the liver in East Asia are HBV carriers. Cheng et al. found that chronic infection with HBV was associated with a higher risk of developing nonclassic RILD (which presented as elevated serum transaminases) in a univariate analysis of patients treated with radiation (Cheng et al. 2002, 2004a). After stratifying their patients, they noted that the HBV carrier group had a shifted normal tissue complication probability curve, with a reduced tolerance compared to the noncarrier group. These arguments suggested the possibility of a unique mechanism (e.g. HBV reactivation) in the development of non-classic RILD (and reduction in liver dose tolerance) in carriers of HBV.
In general, the reactivation of viral hepatitis in HBV carriers can occur without a causative factor as a sporadic event (Tsai et al. 1992). Hepatic venulitis can be seen as part of the picture in viral hepatitis, and other causes of severe hepatitis, leading to significant injury and subsequent loss of affected venules. This could subsequently produce sinusoidal congestion. Because the injury is often patchy, the sinusoidal congestion usually is minimal, as the liver is capable of diverting flow toward adjacent veins with no or milder injury. Chemotherapeutic agents have been associated with HBV reactivation (Yeo et al. 2000). The reactivation of HBV is implicated as the mechanism why HBV patients may have a reduced tolerance to irradiation, though only recently has data been collected to confirm viral reactivation after liver irradiation. In the 2004 Cheng et al. report, 2 of the 17 patients who developed RILD were examined for reactivation of HBV, and both patients demonstrated serologic evidence of viral reactivation (Cheng et al. 2004b). In a separate report by Cheng et al., it was shown that 6 of 8 patients with gastric cancer who developed nonclassic RILD were chronic HBV carriers, and 4 of the 6 carriers had serologic evidence of viral reactivation following adjuvant chemotherapy and radiation therapy (Cheng et al. 2004a).
Kim et al. compared liver irradiation in a group of patients with chronic hepatitis B requiring antiviral treatment at the time of irradiation versus a group of HBV carrier patients without active disease. They found that reactivation of HBV and nonclassic RILD, though not necessarily together, occurred more commonly in the latter group (Kim et al. 2007).
Although it has become established that reactivation of HBV may occurs after liver irradiation, the mechanism is not yet known. Radiation could directly reactivate HBV residing in a hepatocyte, or it may be due to the effect of radiation on nearby nonparenchymal cells (Chou et al. 2007). The direct effect and the so-called bystander effect were tested by Chou et al. by transferring various conditioned media in vitro. They found that the direct effect of radiation on hepatocytes, normal or cancerous, did not show reactivation of HBV. However, transferring conditioned medium of irradiated noncancerous hepatocytes or irradiated endothelial cells resulted in HBV DNA replication (Chou et al. 2007). They went on to show that IL-6 that is released from endothelial cells after irradiation, suggesting that radiation-induced reactivation of viral hepatitis B probably occurs due to a bystander effect of IL-6.
3.3 Biology (Molecular Mechanisms of RILD)
3.3.1 Sinusoidal Endothelial Injury
It has been traditionally postulated that the initiating lesion of VOD in RILD occurs at the level of the sinusoidal endothelium. Electron microscopy studies of affected liver in classic RILD have revealed fibrin deposits throughout the central veins and adjacent sinusoids. These findings led to the supposition that damage to sinusoidal endothelial cells (SECs) and central vein endothelium initiates activation of the coagulation cascade, leading to accumulation of fibrin in the central veins and hepatic sinusoids (Lawrence et al. 1995). It is thought that deposited fibrin serves as a foundation for the proliferation of reticulin and collagen, which eventually occludes the vessel lumen. The trapping of erythrocytes in this collagen meshwork produces vascular congestion and decreased oxygen delivery to the central zone, which is inherently sensitive to hypoxia due to the physiological oxygen gradient within the hepatic lobule. This hypoxic milieu presumably results in death of centrilobular hepatocytes and atrophy of the inner hepatic plate, producing the hepatic dysfunction observed clinically in RILD (Lawrence et al. 1995).
3.3.2 Sinusoidal Obstructive Syndrome
A similar pathological entity to the VOD of classic RILD, named sinusoidal obstructive syndrome (SOS) to distinguish it from other forms of VOD, has been observed in several clinical scenarios. Classic causes of SOS include ingestion of herbal teas or foods containing pyrrolizidine alkaloids, prolonged immunosuppression with azathioprine, and a wide range of chemotherapeutic agents, including dacarbazine, and cyclophosphamide. The most common cause of SOS is myeloablative chemotherapy or combination chemoradiotherapy for bone marrow transplantation (BMT).
Studies of monocrotaline-induced SOS models have delineated a clear sequence of events by which sinusoidal and venous obstruction occurs in rats (DeLeve et al. 1999). In this model, the earliest observed changes appear to be loss of SEC fenestrations and appearance of gaps in SEC endothelium (DeLeve et al. 1999). Digestion of extracellular matrix on the abluminal side of SECs membrane causes SECs to “round up” and facilitates penetration of red blood cells into the underlying space of Disse, leading to dissection of the endothelial lining and narrowing of the sinusoidal lumen (DeLeve et al. 1999, 2003). The dissected sinusoidal endothelium embolizes and obstructs downstream sinusoids and central veins, producing hepatic vascular congestion. Clinical signs of SOS can occur in the absence of central vein occlusion, implying that sinusoidal injury is sufficient to cause clinically significant microcirculatory hepatic dysfunction, as confirmed in this animal model of SOS. By comparison, central vein obstruction is considered a prominent and defining pathological feature of classic RILD, presumably occurring secondary to luminal deposition of fibrin, reticulin, and collagen that traps of erythrocytes and produces vascular congestion and hepatic hypoperfusion.
The earliest morphological change observed in human studies of SOS is edematous widening of the subendothelial space of central and sublobular veins by fragments of erythrocytes (RBCs) and cellular debris (Shulman et al. 1980, 1987, 1994). This is accompanied by sinusoidal dilation and engorgement, erythrocyte penetration into the space of Disse, and necrosis of perivenular hepatocytes (DeLeve et al. 2002). Similar to the animal models, the sinusoidal pathology in SOS is typically more prominent than the central vein changes, an important difference from classic RILD. Sinusoidal injury is thought to be the responsible for the hepatocellular damage and clinical signs of SOS. This hypothesis is supported by findings that clinical signs of SOS correlate with perivenular lesions in the absence of venous occlusion, and that, in one series, 45 % of patients with mild-to-moderate SOS and 25 % of patients with severe disease lacked central vein obstruction on autopsy (Shulman et al. 1994). However, central vein involvement has been shown to correlate with both ascites and disease severity, suggesting that while not requisite to disease development, central vein obstruction significantly exacerbates circulatory impairment and hepatic dysfunction in SOS (Shulman et al. 1994; Rollins 1986).
As in classic RILD, deposition of fibrin and collagen has been observed in SOS, although reports of this finding vary. The fibrosis occurs in the zone-3 sinusoids and can range from mild-to-severe (cirrhotic) depending on the severity and extent of sinusoidal injury. Shulman et al. compared autopsy samples of liver tissue from BMT patients with VOD and without VOD by immunohistochemical analysis (Shulman et al. 1987). Of 11 patients 9 with early VOD demonstrated dense factor VIII staining of central veins, compared to only 2 out of 12 patients without VOD, suggesting activation of the coagulation cascade in the development central vein obstruction in SOS. The observation in this study that positive staining for fibrinogen was present in 4 out of 4 patients with early VOD, compared to 0 out of 4 patients without VOD, supports a role for clotting in the development of early SOS. Furthermore, extensive luminous obliteration by collagen in late VOD was identified in central veins (2/4 pts) and centrilobular sinusoids (3/4 pts), as compared to the localization of collagen in early VOD to only sinusoids (2/3 pts) and not central veins (0/3 pts) (Shulman et al. 1987). Although derived from a small patient population, the temporal differences in these findings between early and late VOD support the theory that sinusoidal endothelial injury initiates the pathogenic cascade in SOS, and that consequent deposition of fibrin serves as a scaffolding upon which collagen accumulates and obstructs sinusoids and downstream central veins.
Other studies of patients undergoing myeloablative BMT (Sartori et al. 2005; Brooks et al. 1970) provide anecdotal evidence for the involvement of clotting cascade activation of the development of SOS. In one study of 53 consecutive pediatric patients receiving BMT, children who developed VOD had significant increases in plasminogen activator inhibitor type I, tissue plasminogen activator, and D-dimer and significant decreases in prothrombin time, antithrombin, and α2-antiplasmin at either 2 days prior to or 1 day after clinical diagnosis of VOD, as compared to patients who did not develop VOD (Sartori et al. 2005). Several retrospective analyses in BMT patients have demonstrated less frequent symptoms and lower incidences of SOS in cohorts receiving prophylaxis therapy with heparin, defibrotide, and/or prostaglandin E1, providing further support for a causal or pathogenic role of thrombosis and hypercoagulability in the development of SOS (Chalandon et al. 2004; Rosenthal et al. 1996; Simon et al. 2001). Given the widespread morphological similarities between classic RILD and SOS, one may speculate that aberrant coagulation cascade activation may also play a role in the pathogenesis of classic RILD, although no clinical studies to date have adequately addressed this possibility.
Despite their pronounced etiological and clinical differences, the morphological similarities between VOD and SOS suggest that SOS may be an important disease model from which we can gain a better understanding of classic RILD. Analogous to the postulated mechanism of RILD, liver sinusoidal endothelial cells appear to be the primary target of drugs that produce SOS (DeLeve 1994, 1996, 1998). Of particular significance is the fact that all of these compounds are (a) detoxified by glutathione (GSH) and (b) selectively more toxic to SECs than to hepatocytes (DeLeve 2007). Depletion of GSH due to metabolism of each of these compounds precedes SEC toxicity in vitro, suggesting that metabolite conjugation by GSH may play a protective role in preventing SEC damage and SOS caused by these drugs (DeLeve 1994, 1996, 1998).
In summary, it is plausible that, as in SOS, damage to SECs may precede the development of central vein obstruction in classic RILD. In SOS, central vein involvement appears to worsen the severity of disease (Shulman et al. 1994; Rollins 1986). It is equally plausible that the scenario is analogous for radiation-induced liver damage, as injury limited to SECs may produce only subclinical disease, whereas obstruction of downstream central and sublobular veins results in clinically manifest RILD.
3.3.3 Activation of Hepatic Stellate Cells
In addition to endothelial cell damage, several other mediators of hepatic damage are thought to contribute to the development of RILD. Hepatic stellate cell (HSC) activation, in particular, has been observed in patients who developed severe congestive changes characteristic of RILD in the months following RT for biliopancreatic cancer, prior to the development of fibrosis (Sempoux et al. 1997). In a study of six such patients by Sempoux and colleagues, activated HSCs, characterized by expression of the α-isoform of smooth muscle actin (α-SMA), were variably identified throughout congested irradiated areas and found within thickened central vein walls and adjacent to or within congested centrilobular sinusoids (Sempoux et al. 1997), while activated HSCs were not present in all samples from all patients and were unevenly distributed in congested areas, their presence did appear to coincide with severity of fibrosis and architectural distortion. α-SMA positive cells were also notably absent from nonirradiated areas, suggesting an association between hepatic irradiation and stellate cell activation. While the small patient population (n = 6) and lack of statistical analysis limits our ability to draw any conclusions from this study, the observations of stellate cell activation in RILD are nonetheless intriguing and warrant further investigation.
3.3.4 Transforming Growth Factor-β
The transforming growth factor-β (TGF-β) family consists of the TGF-β1, TGF-β2, and TGF-β3 precursor isoforms (Blobe et al. 2000; Khalil 1999). The propeptides are cleaved by proteolytic cleavage from the active TGF-β peptide growth factor that exists functionally as a dimer held together by disulfide bonds. TGF-β is ubiquitous as nearly every cell in the human body produces TGF-β and has receptors for it (Blobe et al. 2000). TGF-β is integral in the interaction between cells and the extracellular matrix in the processes of inflammation, immune response, and wound healing. It is also important in cell cycle regulation and in the apoptotic pathway. The reactive interaction between parenchymal cells, nonparenchymal cells, and the extracellular environment occurs through the language of cytokines. TGF-β proves to be one of the most important cytokines associated with tissue injury and wound healing (Amento and Beck 1991; Barcellos-Hoff 2005a, b; Barcellos-Hoff et al. 2005). The pathogenesis of RILD, though still unclear, may have less to do with direct parenchymal damage and more likely is a result of the tumor microenvironment as a result of irradiation.
As such, TGF-β has been implicated in playing a role in the fibrogenesis leading up to RILD. Fibrin deposition in the sinusoids and subendothelial space has been shown to occur after liver irradiation (Fajardo and Colby 1980). After a stable period of fibrin deposits, fibroblasts migrate to the region of injury and undergo proliferation and production of collagen under the influence of TGF-β (Amento and Beck 1991). TGF-β is not necessarily associated with radiation injury; rather, it is present in a non-specific manner in many types of liver disease, such as cirrhosis, chronic hepatitis, or chemotherapy-induced liver disease (Anscher et al. 1993; Castilla et al. 1991).
Anscher et al. described the dose dependence of the level of TGF-β in irradiated liver; they also showed that the level of TGF-β in hepatocytes was higher in the region of more extensive fibrosis (Anscher et al. 1990). After creating an association with TGF-β, dose, and fibrosis, they were able to identify a temporal or cause-and-effect relationship by demonstrating a fibrotic reaction in healthy rat livers after injection with TGF-β (Anscher et al. 1990). Rodent models of VOD and RILD have been elusive (as described in Sect. 3.2.3). Anscher and colleagues reported that the level of TGF-β in normal human hepatocytes was higher than that in normal rat hepatocytes, raising a possible reason why humans have a greater tendency to develop VOD as compared to rats (Anscher et al. 1990). The same group reported separately that patients who received induction chemotherapy prior to BMT and high-dose chemotherapy and developed VOD after treatment had significantly higher levels of pretransplantation TGF-β as compared to patients who did not develop VOD and to normal controls (Anscher et al. 1993).
TGF-β may not be the only factor contributing the mechanism of RILD, but it is certainly significant in the pathogenesis. Furthermore, it is a compelling hypothesis that TGF-β may play a role in determining why humans develop VOD more readily than other animal models.
3.3.5 Other Cytokines
Aside from TGF-β, there are a number of other cytokines and downstream reactants that participate in the response to tissue injury. The inflammatory condition that leads to cytokine release is typically associated with injury (e.g. burns, trauma), exposure to endotoxins, or exposure to foreign materials (e.g. infection). The importance of the inflammatory response in these conditions is in recruiting leukocytes to the site of stress so that the host may react to the damage. Gourmelon et al. has shown that a similar cytokine release pattern occurs in nonhuman primates subsequent to whole body irradiation (Gourmelon et al. 2005). A number of authors have similarly demonstrated the release of inflammatory cytokines with irradiation of various organs or cell types (Fedorocko et al. 2002; Linard et al. 2003; Beetz et al. 1997; Meeren et al. 1997; Hosoi et al. 2001; Ishihara et al. 1993).
Similarly, Christiansen et al. showed that irradiation of rat liver in vivo induces upregulation of proinflammatory cytokines IL-1-beta, IL-6, and tumor necrosis factor alpha (Christiansen et al. 2007). The same group demonstrated that in vitro irradiation of isolated hepatocytes alone showed no effect versus irradiation of hepatocytes in a medium supplemented with the proinflammatory cytokines, which induced the upregulation of hepcidin, an acute phase reactant (APR). Moriconi et al. were able to show that hepatocytes had upregulation of chemokines after irradiation in vivo (Moriconi et al. 2008). Similarly, they showed that in vitro hepatocytes had upregulation of these chemokines only in the presence of proinflammatory cytokines. Although higher dose tolerance has been shown for partial liver irradiation (Dawson and Ten Haken 2005), the whole liver is sensitive to irradiation, with greater than a 5 % risk of toxicity above doses of 30–35 Gy in 2-Gy fractions (Ingold et al. 1965; Emami et al. 1991; Austin-Seymour et al. 1986). Conversely, hepatocytes irradiated in vitro have been demonstrated to be relatively radioresistant (Jirtle et al. 1984; Jirtle et al. 1982; Jirtle et al. 1981; Alati et al. 1988, 1989a, b). Therefore, hepatocytes irradiated in vivo or in a medium of proinflammatory cytokines demonstrate a weakened state that confers the overall radiosensitivity of the whole liver. The proinflammatory cytokines are not released from the hepatocytes themselves, but are released from nonparenchymal cells (i.e. Kupffer cells and SECs) and via a paracrine affect can induce hepatocyte production of APRs (Christiansen et al. 2007; Moriconi et al. 2008; Ramadori and Armbrust 2001).
In other types of liver injury, chemokines play a central role in the recruitment of leukocytes (Ley 1996; Mehendale 2005), which likely contribute to the development of fibrosis. However, despite the upregulation of chemokines from hepatocytes after irradiation, Moriconi et al. did not observe significant leukocyte recruitment in the liver (Moriconi et al. 2008).
4 Clinical Syndromes
4.1 Clinical Endpoints
A variety of endpoints characterize radiation-induced liver injury. It is sometimes useful to categorize the endpoints, albeit somewhat arbitrarily, as shown in Table 2. Note that the cytokine changes have been demonstrated primarily in preclinical models, and are not ready to be used routinely in a clinical setting.
4.1.1 Liver Toxicity Subtypes
Liver toxicity has not always been separated into “classic” and “non-classic” RILD in the literature, which has contributed to some challenges in understanding the different pathophysiology and the dependence on radiation dose and volume for different liver toxicities that may occur following irradiation. Below, potential liver toxicities that may occur following liver irradiation are described. Of note, “non-classic RILD” includes any serious liver toxicity that may not fit into the other categories, and with time it is possible that different nonclassic RILD toxicities may be better understood and separated as entities of their own.
4.1.1.1 Classic RILD
As described previously, the most commonly studied hepatic toxicity following liver irradiation is classic RILD, a clinical syndrome mimicking VOD following stem cell transplantation, presenting with anicteric ascites and hepatosplenomegaly (Lawrence et al. 1995). RILD typically occurs between 2 weeks and 3 months after the completion of irradiation. Patients typically present with fatigue, rapid weight gain, increased abdominal girth, and, occasionally, right upper quadrant pain or discomfort, in the absence of icterus or jaundice. Ascites and hepatomegaly may be seen on physical examination. Laboratory findings usually consist of significant elevations in alkaline phosphatase (3–10 times upper limit of normal) and mildly increased aspartate transaminase (AST) and alanine transaminase (ALT) levels (~2 times upper limit of normal), with other liver function tests, including bilirubin, within normal limits (or at baseline pre treatment levels). RILD is defined in the absence of intrahepatic progression of cancer, as progressive cancer may mimic or mask RILD.
The clinical manifestations and course of RILD differ significantly from that of acute liver failure. Patients in acute liver failure typically present with more acute symptoms of nausea, vomiting, abdominal pain, dehydration, and jaundice. While RILD can progress to fulminant hepatic failure, particularly in the presence of underlying hepatic dysfunction, patients rarely present in acute liver failure. Figure 6 outlines the clinical workup, differential diagnosis, and management of classic RILD.
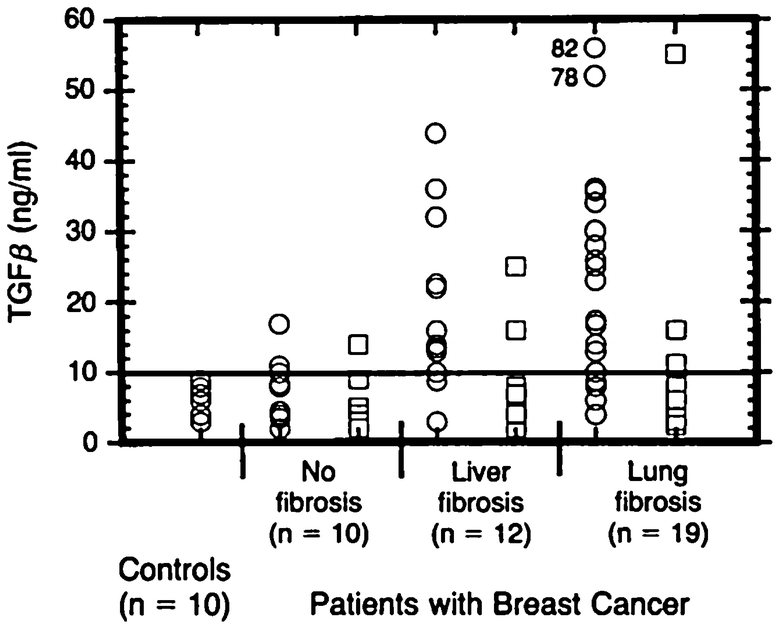
Fig. 6
Transforming growth factor β as a predictor of liver and lung fibrosis after autologous bone marrow transplantation for advanced breast cancer by Anscher et al. (1993). Individual TGFβ plasma concentrations in the four study groups. Healthy blood donors served as controls. One roup of patients did not have pulmonary fibrosis or hepatic veno-occlusive disease after high-dose chemotherapy and autologous bone marrow transplantation (no fibrosis); the two other groups had either hepatic veno-occlusive disease (liver fibrosis) or pulmonary fibrosis (lung fibrosis). TGFβ was measured before (circles) and after (squares) high-dose chemotherapy and transplantation (Anscher et al. (1993) shows the timing of the regimens). The solid horizontal line indicates the value (10 ng per milliliter, or 2 SD above the mean value determined in the controls) that was used as a cutoff point to determine the ability of pretransplantation TGFβ measurement to predict the development of hepatic or pulmonary toxicity after transplantation. To convert values for TGFβ to millimoles per liter, multiply by 4 × 10−8 (with permission from Anscher et al. 1993)
4.1.1.2 Nonclassic RILD
Nonclassic RILD is defined as elevated liver transaminases more than five times the upper limit of normal or CTCAE grade 4 levels in patients with baseline values more than five times the upper limit of normal within 3 months after completion of RT or a decline in liver function (measured by a worsening of Child-Pugh score by 2 or more), in the absence of classic RILD or progression of intrahepatic cancer (Pan et al. 2008). This endpoint has been described most commonly in patients with hepatocellular carcinoma who have compromised baseline liver function (hepatitis B infection, Child-Pugh B or C liver function) (Cheng et al. 2003; Liang et al. 2006).
A confounder of classic and nonclassic RILD, especially in populations with pre-existing liver dysfunction, is the baseline decline in liver function within this population that occurs with time due to pre-existing liver disease and/or progressive liver cancer. In a randomized trial in unresectable hepatocellular carcinoma of placebo versus Sorafenib, a multitarget kinase inhbitor, there was a 54 % rate of serious adverse events among the placebo group due to progression of cirrhosis and/or cancer (Llovet et al. 2008). Nontheless, liver function (e.g. Child-Pugh score) is an important endpoint to report following treatment, as a decline in liver function is clinically relevant, whether it is due to progressive liver cancer, underlying cirrhosis or treatment. Thus, in addition to reporting nonclassic RILD in patients without progression of liver disease, any change in liver function (as measured using Child-Pugh score, Table 1) at 3 months following irradiation is recommended to be reported in all patients irradiated, including patients with progressive disease.
Table 1
Child-Pugh scoring system to assess severity of liver disease or toxicity
Criterion
|
1 point
|
2 points
|
3 points
|
---|---|---|---|
Bilirubin (total)
|
<2 mg/dL
|
2–3 mg/dL
|
>3 mg/dL
|
Albumin
|
>3.5 g/dL
|
2.8–3.5 g/dL
|
<2.8 g/dL
|
INR
|
<1.7
|
1.71–2.20
|
>2.20
|
Ascites
|
None
|
Controlled with medication
|
Refractory
|
Hepatic encephalopathy
|
None
|
CTCAE grade I–II (or controlled with medication)
|
CTCAE grade III–IV (or refractory)
|
Points
|
Class
|
2-year survival (%)
|
---|---|---|
5–6
|
A
|
85
|
7–9
|
B
|
57
|
10–15
|
C
|
35
|
Table 2
Representative endpoints of radiation-induced liver injury segregated as clinical vs. subclinical and global vs. focal, as shown
Focal
|
Global
|
|
---|---|---|
Subclinical
|
Regional changes on contrast CT
Regional changes on contrast MR
Regional changes in MR SPECT
|
Ascites
Hepatosplenomegaly
Increased ALP
Increased liver enzymes (ALT, ALP)
Increased Hepatitis B antigen
Thrombocytopenia
Changes in albumin, bilirubin, and INR
Changes in indocyanine green (ICG) extraction
Elevated TGF-β
Elevated IL-1β
Elevated IL-6
Elevated TNF alpha
Elevated N-terminal peptide of type III procollagen propeptide
Reduced protein C
|
Clinical
|
Fibrosis in critical region of liver, e.g., common bile duct leading to biliary obstruction
|
Increased abdominal girth
Right upper quadrant pain
Weight gain
Fatigue
Edema
Confusion
Encephalopathy
|
4.1.1.3 Reactivation of Hepatitis B Virus
Some patients who develop elevation of their liver enzymes, as described in Sect. 4.1.2, likely had hepatitis B reactivation induced by irradiation (Cheng et al. 2004a). Reactivation of HBV has now been described following liver irradiation in animal models and in patients. Reactivation of HBV can occur following chemotherapy, and thus, it is likely more common following combined chemotherapy and radiation in HBV carriers.
Patients planned to be treated with radiation therapy should be considered for tested for hepatitis B surface antigen (HBsAg) and anti-HCV. If either test is positive, further confirmatory testing should be done including HBV DNA or HCV RNA, and a referral to a hepatologist is recommended for treatment of viral hepatitis prior to irradiation.
4.1.1.4 Post Transplant Veno-Occlusive Disease
VOD is a frequent, serious, and often life-threatening complication of hematopoietic stem cell or bone marrow transplantation. It is believed to be caused by hepatic endothelial cell injury from the high-dose cytoreductive conditioning regimens used during stem cell and bone marrow transplant protocols, often in combination with total body irradiation (TBI) (DeLeve et al. 2002; Bearman et al. 1993). Hepatocytes in zone 3 of the liver acinus are also injured by direct chemotherapy toxicity and ischemia due to endothelial clotting in the terminal hepatic venules.
Patients present with hyperbilirubinemia, fluid retention, and hepatomegaly. VOD has been defined as bilirubin ≥2.0 mg/dL, weight gain ≥2.5 % from pretransplant weight, and hepatomegaly and/or right upper quadrant pain, occurring within 2 weeks of transplantation, in the absence of other explanations for these signs or symptoms. The primary difference between transplant-associated VOD and RILD is the rapid elevation of bilirubin which may occur in transplant VOD within days following chemotherapy or TBI. Risk factors for VOD include previous hepatitis, mismatched or unrelated donor transplants, and high-dose chemotherapy regimens (i.e. high-dose Cyclophosphamide, or Cyclophosphamide and Busulfan). Interestingly, the use of TBI and the doses used in preparative regimens do not appear to be strongly associated with the risk of VOD occurring (Ganem et al. 1988).
VOD occurs in up to 50 % of patients following stem cell transplantation. 50 % of cases are irreversible and can lead to multiorgan failure. Disease prognosis generally depends on the degree of liver injury and the resulting severity of the hepatic dysfunction. Mild disease may not develop liver failure, and may reverse with supportive treatment. Moderate VOD includes evidence of liver dysfunction, and treatment with diuretics for fluid retention, and analgesics for right upper quadrant pain are often required. Fifty percent of patients present with severe and life-threatening VOD. These patients have the most dramatic rises in serum bilirubin, the fastest weight gain, the highest rates of ascites, and the most severe hepatic dysfunction. Prevention of VOD with heparin, prostaglandin E, and ursodeoxycholic acid have not definitively been shown to prevent or mitigate transplant-related VOD. Treatment with supportive care does not usually alter the course of this toxicity, and these patients often die of severe renal and cardiac failure. The day +100 post stem cell transplant mortality for these patients approaches 100 % (Bearman et al. 1993).
4.1.2 Grading of Toxicity
The Cancer Therapy Evaluation Program, Common Terminology Criteria for Adverse Events (CTCAE), Version 3.0, grades hepatobiliary toxicity according to clinical criteria including jaundice, asterixis, and encephalopathy or coma for grades 2, 3, and 4, respectively. These serious adverse events are rare following radiation therapy. Acute changes in liver enzymes or liver function tests are far more common during and/or following radiation therapy. Such liver enzyme abnormalities are classified under the CTCAE metabolic/laboratory category.
The Child-Pugh scoring system to assess liver dysfunction is based on an assessment of the clinical and laboratory parameters shown in Table 1. It can be used to characterize baseline liver function and also post-treatment changes in liver function.
4.2 Detection (Liver Function Tests)
4.2.1 Serum Markers
Assessment of liver function is an important component of evaluation of patient with liver cancer or other cancers in whom radiation therapy may be used for treatment. Serial measures of liver function are also useful in estimating the severity of radiation toxicity, and in predicting survival following development of classic or non-classic RILD. Hepatic synthetic function is assessed by measurement of serum albumin, bilirubin, and prothrombin time, which are critical components of the Child-Pugh scoring system. Decrease in platelet count and white blood cell counts may reflect the degree of portal hypertension and associated hypersplenism.
Other tests such as isocyanine green retention and 99m-Tc GSA (diethylenetriamine-penta-acetic acid-galactosyl human serum albumin) scintigraphy have been described as more specific indicators of hepatic reserve in preparation for resection, but have not convincingly surpassed the Child-Pugh classification as a predictor of post-operative complications and liver failure.
4.2.2 Indocyanine Green Clearance
The pathophysiology of RILD, as described in Sect. 3, leads to portal venous congestion and thereby altered vascular outflow from the liver. Cao et al. concluded that hepatocyte function was altered in the area of hypoperfusion using an indocyanine green (ICG) extraction study (Cao et al. 2008). While ICG clearance has been used as a measure of liver function, there have been discrepancies when compared with histological findings. ICG is taken up from plasma almost exclusively by hepatocytes, and subsequently secreted into bile. However, this function of hepatocytes does not measure the synthetic function or conjugative abilities of hepatocytes. Furthermore, ICG analysis measures whole liver function, and it is unable to identify specific regions of diminished functionality. Therefore, the outcome of the ICG test ultimately defines whether perfusion of the whole liver is adequate, where functionality of one portion of the liver can overshadow the dysfunction of another part of the liver. A more meaningful analysis would be to measure the function of the irradiated portion of the liver.
4.3 Diagnosis (Imaging)
Focal changes in irradiated liver were documented on scintillation scanning as early as 1967 (Concannon et al. 1967). Changes in computed tomographic (CT) and MRI contrast enhancement have been seen within regions of the liver irradiated to high doses (Herfarth et al. 2003). Ultimately, the liver volume irradiated to high doses becomes fibrotic while the spared liver hypertrophies, as shown in Fig. 7. The threshold doses for these effects is a topic of ongoing research.
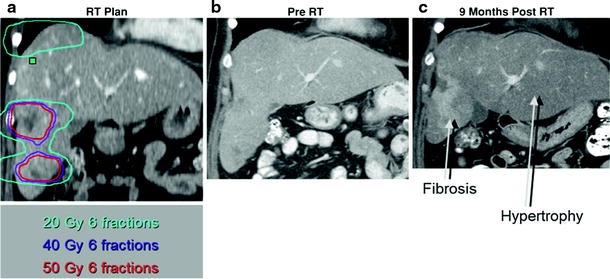
Fig. 7
Coronal views of preradiation therapy CT scans, with a and without b the radiation dose distribution shown, of a patient with multiple liver metastases, compared to CT 9 months following radiation therapy c, with fibrosis occurring in the liver irradiated to high doses and hypertrophy of the spared left lobe
Characterizing RILD by noninvasive imaging has been challenging and the techniques are evolving. The CT findings of RILD were first described in a report of three patients who received partial liver irradiation (Jeffrey et al. 1980). The post-irradiation CTs showed low density of the irradiated portion of liver, sharply demarcated from the liver outside of the radiation ports. On follow-up CT scans between 4 and 14 months after radiation therapy, the low-density band had resolved and liver function was returned to normal unless affected by progression of the primary cancer (Jeffrey et al. 1980).
Unger et al. later described the CT and MR imaging findings of two cases reports of RILD (Unger et al. 1987). This group confirmed the CT findings of low attenuation in the portion of irradiated liver, again sharply demarcated from unirradiated liver. This area of low density could represent increased water or fat content. An MR of the same patients showed decreased signal intensity on T1-weighted images, increased signal intensity on T2-weighted images, and increased signal intensity on proton spectroscopic imaging in the portion of liver corresponding to the low attenuation on CT, indicating that the irradiated liver consisted of increased water content. On follow-up CTs, there was resolution of the low attenuation portion of liver, and shrinkage of the irradiated liver segment (Unger et al. 1987).
As seen above, CT and MR imaging provides anatomical data in RILD. Unger et al. also performed CT angiography on one patient and found that the liver in the radiation ports demonstrated delayed filling of contrast as compared to unirradiated liver, alluding to the outflow congestion pattern found on pathology (Unger et al. 1987). This was the first step toward identifying the perfusion changes of the liver as it relates to RILD.
Noninvasive CT imaging and the estimation of blood flow by the gradient method was first described for the kidney (Peters et al. 1987a, b). The quantification of blood flow was later described in a mathematical model using information obtained from dynamic CT and read on a color scale (Miles 1991; Miles et al. 1991). Although some groups have used the gradient method to measure liver perfusion (Miles et al. 1993; Blomley et al. 1995; Bader et al. 1998), the liver poses a unique challenge given that it has dual vascular input from the hepatic artery and the portal vein. Materne et al. first developed and validated a method to quantify liver perfusion using the “dual-input, one-compartment” model to account for the dual inflow (Materne et al. 2000).
The finding of VOD in RILD implies that the vascular outflow will be altered, namely in a post-hepatic obstruction manner beginning at the level of the central veins and further distally to the hepatic veins. CT perfusion is an imaging technique that can identify and quantify this vascular flow aberrancy (Cao et al. 2008).
MR single-photon emission computerized tomography (SPECT) has been used to measure asialoglycoprotein receptor (ASGPR). ASGPRs are receptors found in abundance on the sinusoidal surface of hepatocytes that mediate the removal of serum glycoproteins, lipoproteins, immunoglobulin A, fibronectin, and apoptotic cells. They, therefore, can serve as a surrogate for hepatic function. Iguchi et al. (2003) have shown that ASGPR SPECT correlates well with liver fibrosis (Hoefs et al. 2006).
5 Radiation Tolerance and Predicting Liver Toxicity
5.1 Clinical Parameters
Pre-existing liver dysfunction makes patients more susceptible to radiation-induced liver injury, as summarized in Table 3. Patients with Child-Pugh B or C scores have a higher risk of radiation-associated liver toxicity than those with Child-Pugh A scores (Liang et al. 2006; Hata et al. 2006; Ten Haken et al. 2006). Additional risk factors include hepatitis B carrier status (Cheng et al. 2004a), prior transarterial chemoembolization (TACE) (Liang et al. 2006), concurrent chemotherapy (Dawson et al. 2002), portal vein tumor thrombosis (Kim et al. 2007; Liang et al. 2006; Seong et al. 2003), tumor stage (Liang et al. 2006), male sex (Dawson et al. 2002), and cancer of the liver Italian program (CLIP) staging system (Liang et al. 2006; Seong et al. 2003, 2007).
Table 3
SOMA grading system for liver injury
Liver
|
||||
---|---|---|---|---|
Grade 1
|
Grade 2
|
Grade 3
|
Grade 4
|
|
Subjective
|
||||
Pain RUQ
|
Occasional and minimal
|
Intermittent and tolerable
|
Peristent and intense
|
Refractory and excruciating
|
Objective
|
||||
Abdominal findings
Edema
Weight gain
Alertness
Bleeding
|
Hepatomegaly
Occasional leg edema
|
Soft ascites
Intermittent leg edema
≤5 %
Change in attentiveness and sleep pattern
|
Tense ascites
Anasarca responsive to diuretics
>5–10 %
Confusion
Correctable
|
Anasarca unresponsive to diuretics
>10 %
Coma
Unresponsive
|
Management
|
||||
Pain
Abdominal findings
Bleeding
|
Occasional non-narcotic
|
Regular non-narcotic
Intermittent diuretics
Iron therapy
|
Regular narcotic
Permanent diuretics
Occasional transfusion of fresh frozen plasma
|
Continuous narcotic
Frequent transfusions
|
Analytic
|
||||
AST/ALT/Alk phos
|
<2.5 × normal
|
2.5–5.0 × normal
|
>5.0–20.0 × normal
|
>20.0 × normal
|
Bilirubin
|
<1.5 × normal
|
1.5–5.0 × normal
|
>5.0–10.0 × normal
|
>10.0 × normal
|
PT/PTT
|
<1.25 × normal
|
1.25–1.5 × normal
|
>1.50–2.0 × normal
|
>2.0 × normal
|
Serum alb (gm/dl)3
|
>3.0
|
>2.5–3.0
|
>2.0–2.5
|
≤2.0
|
Platelets (1,000)
|
>75.0
|
>50.0–75.0
|
>25.0–50.0
|
≤25.0
|
As a measure of baseline liver reserve, baseline indocyanine green retention rate at 15 min was investigated and found to correlate with hepatic insufficiency following proton ion therapy for 30 patients with hepatocellular carcinoma (Kawashima et al. 2005). Eight patients developed hepatic insufficiency, presenting as anicteric ascites, elevated transaminases and/or asterixis, 1–4 months following therapy. No hepatic insufficiency was observed if the retention rate was less than 20 %, whereas 3 of 4 patients with retention rates greater than 50 % developed hepatic insufficiency.
The tolerance of the liver to whole organ irradiation does not appear to be substantially altered by the concomitant use of fluoropyrimidines. In contrast, whole liver irradiation in combination with alkylating agents or mitomycin C is associated with an increased risk of liver toxicity (Lawrence et al. 1995; Haddad et al. 1983; Schacter et al. 1986). In one study investigating the partial volume tolerance of the liver to irradiation, the use of concurrent hepatic arterial bromodeoxyuridine (BUdR) chemotherapy increased the risk of RILD compared to concurrent hepatic arterial fluorodeoxyuridine (FUdR) (Dawson et al. 2002).
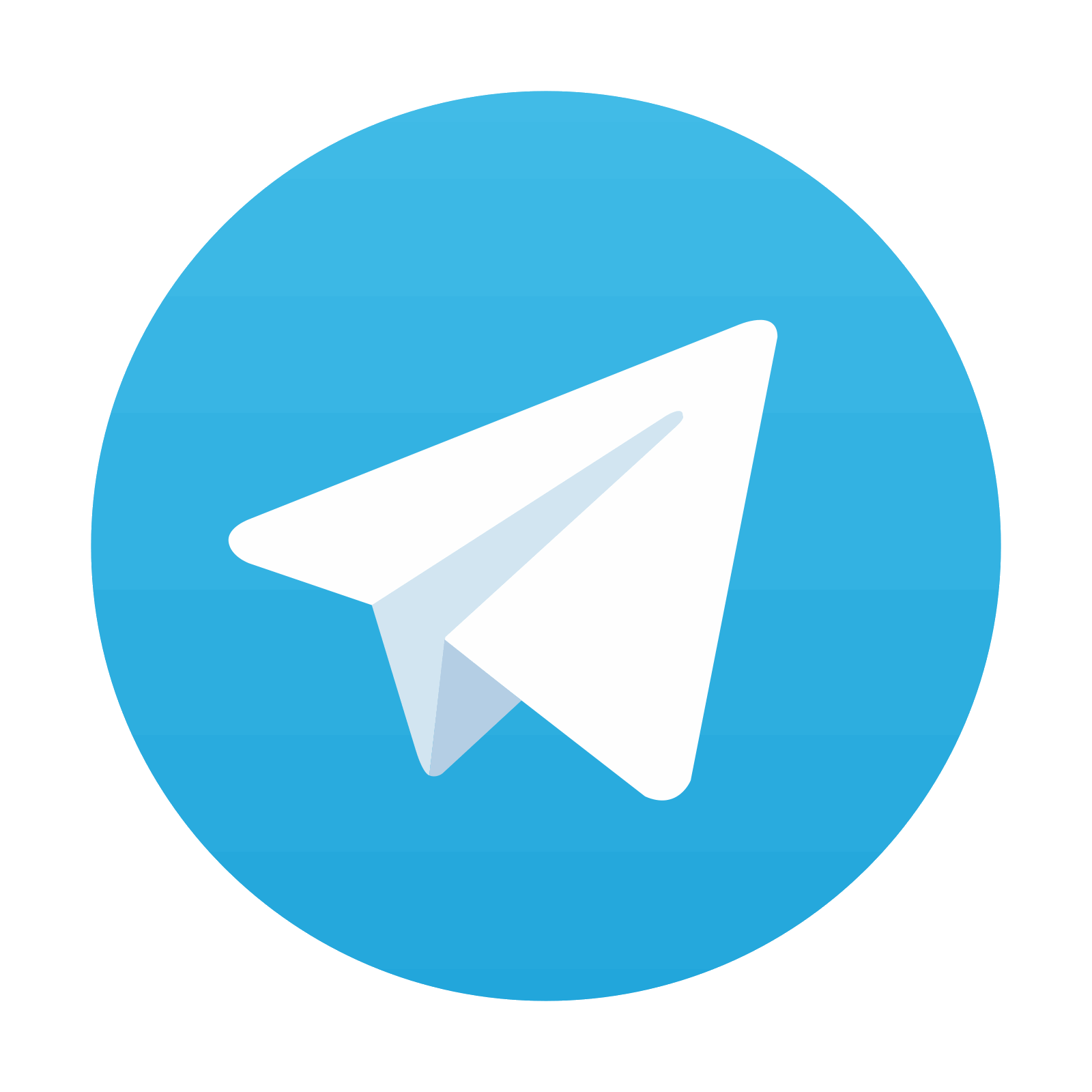
Stay updated, free articles. Join our Telegram channel
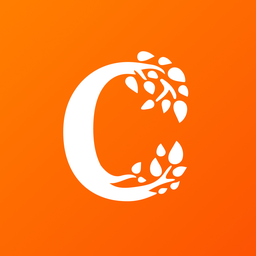
Full access? Get Clinical Tree
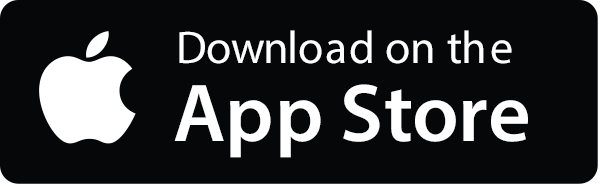
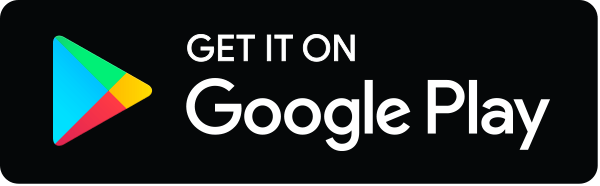