Although traditional catheter-based angiography has been the gold standard for pediatric abdominal and pelvic vascular imaging for the past several decades, advances in magnetic resonance angiography (MRA) have made it a viable alternative. MRA offers several advantages in that it is noninvasive, can be performed without ionizing radiation, and does not necessarily rely on contrast administration. The ability of modern MRA techniques to define variant vascular anatomy and detect vascular disease may obviate traditional angiography in some patients.
Key points
- •
Magnetic resonance angiography is particularly well suited for pediatric abdominal and pelvic vascular imaging because of its noninvasive nature and lack of ionizing radiation.
- •
The lack of a gadolinium-based contrast agent approved by the US Food and Drug Administration, contrast dosage restrictions, and the potential difficulty of obtaining reliable venous access render noncontrast magnetic resonance angiography a desirable modality in pediatric patients.
- •
Contrast-enhanced magnetic resonance imaging provides the ability to evaluate vascular anatomy and disease in multiple phases (ie, arterial, portal venous, and systemic venous phases).
- •
MR angiography allows for identification of various pathologic conditions and anatomic variants in the arterial, systemic venous, and portal venous systems, precluding, in some cases, the need for gold-standard catheter-based angiography.
Introduction
Advances in magnetic resonance (MR) scanner technology and pulse sequences have made magnetic resonance angiography (MRA) a clinical reality for pediatric abdominal and pelvic vascular imaging. Compared with computed tomography (CT) techniques for imaging the cardiovascular system, MRA offers several advantages, including native multiplanar capabilities, superior tissue contrast, and noncontrast angiographic imaging. However, it is the lack of ionizing radiation and its noninvasive nature that make MRA such a compelling technique for pediatric use. The first half of this article focuses on the technique of obtaining high-quality MRA and the second half highlights anatomic variants and vascular diseases that can be identified in the arterial, venous, and portal venous systems.
Introduction
Advances in magnetic resonance (MR) scanner technology and pulse sequences have made magnetic resonance angiography (MRA) a clinical reality for pediatric abdominal and pelvic vascular imaging. Compared with computed tomography (CT) techniques for imaging the cardiovascular system, MRA offers several advantages, including native multiplanar capabilities, superior tissue contrast, and noncontrast angiographic imaging. However, it is the lack of ionizing radiation and its noninvasive nature that make MRA such a compelling technique for pediatric use. The first half of this article focuses on the technique of obtaining high-quality MRA and the second half highlights anatomic variants and vascular diseases that can be identified in the arterial, venous, and portal venous systems.
Technique
Pediatric MRA Protocol Considerations
There is no single correct methodology for performing MRA in the pediatric patient population. Instead, a menu of different techniques exists for radiologists to optimize angiographic evaluation in order to answer the clinical question. Broadly, these techniques can be broken into 2 basic groups: noncontrast sequences and contrast-enhanced (CE) techniques ( Table 1 ). The advent of CE-MRA techniques over the past 2 decades has largely replaced traditional noncontrast techniques for imaging most vascular territories. The marked increase in signal provided by gadolinium-based contrast agents (GBCA) allows MRA to be performed rapidly, typically covering a large field of view in a single breath hold. In addition, the high signal obtained using CE-MRA allows for high spatial resolution, which often aids in depiction of subtle vascular wall abnormalities or peripheral vascular branches. Because of the high spatial resolution, a single three-dimensional (3D) data set can be obtained with CE-MRA that allows reconstruction of oblique imaging planes, along with other postprocessing techniques. Although the advantages of CE-MRA were recognized early in adult populations, this technique has now also become standard in the pediatric population. Nonetheless, there has also been a rejuvenated interest in modern noncontrast MRA techniques over the past several years.
Noncontrast | Black blood Time of flight Phase contrast Steady-state free precession |
Contrast enhanced | Multiphase dynamic postcontrast imaging Bolus tracking or timed MRA Keyhole MRA |
Central to this renewed interest in noncontrast imaging is the recognition of an association between GBCA and nephrogenic systemic fibrosis (NSF) in patients with renal dysfunction. NSF is a rare condition characterized by progressive fibrosis and swelling in the extremities of affected individuals, primarily occurring in the skin and integument. In some individuals, the fibrotic process can be progressive, affecting visceral organs such as the lungs, esophagus, and heart, resulting in substantial morbidity or even mortality. CE-MRA is avoided in patients who have renal impairment, such as end-stage renal disease requiring dialysis, severe chronic kidney disease (estimated glomerular filtration rate [eGFR] <30 mL/min/1.73 m 2 ), or acute kidney injury. More recently, patients who show borderline renal function (eGFR 30–40 mL/min/1.73 m 2 ) are increasingly urged to avoid GBCA exposure if possible. Although patients with chronic renal disease are encountered more frequently in the adult population, many pediatric disease processes can present with chronic diminished renal function or more acute kidney dysfunction.
Beyond NSF, there are several other reasons that noncontrast MRA is useful in the pediatric population. No gadolinium contrast agent is approved by the US Food and Drug Administration (FDA) for first-pass MRA in children, so the administration of contrast agents is considered off-label use. In addition, dosing for GBCA is typically achieved using a weight-based dosing scheme, typically 0.1 mmol/kg for standard doses and 0.2 mmol/kg for double doses. Given the physical size of infants and children, this dosing can result in a small amount of injected contrast material, which makes accurate bolus tracking/timing challenging when CE-MRA is used. Reliable venous access in a tiny pediatric patient can be challenging because of the small blood vessel size. The ability to hold the breath for 20 to 30 seconds is commonplace in adults and adolescents, allowing motion-free CE-MRA images to be obtained. However, in children and infants, breath holding is not possible without the use of general anesthesia and mechanical ventilation, thereby adding substantial periprocedural complexity and the risk of sedation/anesthesia. On the other hand, noncontrast MRA techniques can be accomplished with respiratory compensation (ie, navigator pulses or mechanical respiratory tracking with a bellow) and may be more easily tolerated by pediatric patients than full anesthesia.
Noncontrast MRA
Black blood imaging
Black blood imaging is an MRA technique that uses several inversion pulses to nullify the signal from flowing blood. An initial nonselective inversion pulse is applied broadly, followed by a section-selective inversion pulse to the area of interest, which results in signal from static tissues in the slice of interest and lack of signal from moving blood. This situation results in a black appearance of flowing blood, most pronounced in flow that is directly perpendicular to the imaging plane. Black blood sequences are generally preferred in the evaluation of vasculitis, because wall edema/inflammation can be shown directly. The use of cardiac gating can substantially improve overall image quality by reducing pulsation artifacts. Black blood techniques are generally excellent for the depiction of aortic dissection flaps or intramural hematoma, which show excellent tissue contrast versus the nullified signal in the vascular lumen.
Time of flight
Time-of-flight (TOF) MRA is a commonly used noncontrast MRA, which offers unique information relative to CE-MRA because of its directional depiction of blood flow. TOF MRA uses several radiofrequency (RF) pulses to nullify the signal of stationary tissues within a section of interest and subsequently relies on inflowing blood to provide nonsaturated protons, which generate signal for the imaging section. In addition, user-defined saturation slabs can be applied adjacent to the imaging section to purposefully eliminate flow from blood coursing into the slice from a specific direction. Therefore, when applied superior to an imaging slice, this technique causes downward flowing blood to lose signal. This situation can be helpful in identifying flow direction and results in specific arteriographic or venographic depiction. The major limitation of TOF imaging is the long scan times, particularly for large coverage areas such as the extremity circulation. In addition, TOF MRA works best with flow that is directed perpendicular to the imaging plane/section. TOF MRA suffers from signal loss when flow directionality is parallel or within the imaging section, which can artifactually simulate a narrowing or occlusion.
Phase contrast
Phase contrast (PC) MRA is a unique MRA sequence that relies on the phase shift caused by moving blood when placed in a magnetic field. PC MRA results in 2 sets of images, including a magnitude image set (luminal depiction) and a phase image set (velocity/direction depiction). As a result of these 2 data sets, PC MRA can quantify the flow velocity within an imaging section. By specifying the velocity encoding factor that establishes the maximum flow rate for the imaging section, the resulting PC MRA images can be interpreted quantitatively. The ability to interrogate the imaging data for quantifiable flow is useful in patients with vascular shunts (such as a patent ductus arteriosus) and vascular stenosis. PC MRA is time consuming and sensitive to disturbances in flow, resulting in overestimation of stenosis if there is substantial local flow turbulence. Incorrect assignment of the velocity encoding parameter can result in erroneous flow data for velocities higher than this threshold. This situation results in aliasing on the obtained phase images, which may be a clue to the low velocity encoding setting.
Steady-state free precession imaging
Steady-state free precession (SSFP) imaging can be used to generate bright blood MRA images. This gradient echo sequence uses RF pulses to maintain steady-state longitudinal and transverse magnetization. The resultant image has a mixed image contrast, which is based on the T2/T1 ratios, with excellent signal to noise, leading to high signal intensity in both arteries and veins. With modern advances in scanner hardware, SSFP images can be obtained rapidly, which makes it an ideal solution for imaging the uncooperative or nonstationary pediatric patient. SSFP techniques are highly sensitive to field inhomogeneities, which can cause substantial artifact at natural areas of susceptibility, such as air interfaces. In addition, there is relatively poor background suppression for SSFP imaging, because of the image contrast. Therefore, if pure angiographic depiction is desired, the sequence must be modified with preparatory pulses to nullify background tissue.
Future directions
Several other new and emerging noncontrast techniques have been described for body MRA in adult patients, with potential usefulness in children. As an example, electrocardiography-gated fast spin echo (FSE) imaging has been introduced, which uses the difference between arterial signal on FSE imaging during systole and diastole to generate an arteriographic image. In diastole, arterial flow is generally high signal intensity on T2-weighted images, because of the slow flow during this portion of the cardiac cycle. However, arterial flow during systole generally manifests as a flow void on T2-weighted images, whereas other background signal remains similar to diastole. Using image manipulation/subtraction between systolic and diastolic phase images, the arterial signal can be isolated from the data set. This technique allows depiction of flow parallel to the imaging plane, so nonaxial acquisitions may be used to increase imaging speed. Arterial spin labeling is another technique, in which arterial blood is tagged through a set of pulse sequences such that flowing blood is highlighted against a relatively nulled background. Given the success of these techniques in imaging small vessels in adults (such as the renal and coronary arteries), their use in pediatric body MRA seems to be a natural extension.
CE-MRA
The use of GBCA has substantially expanded the use of MRA in clinical applications. Gadolinium chelates alter the local magnetic microenvironment around them, resulting in shortened T1 relaxation times, which translate into faster longitudinal recovery. By using T1-weighted imaging sequences, high vascular signal intensity is achieved with CE imaging. Modern MR scanners use ultrafast 3D T1-weighted gradient echo sequences, often with fat suppression, to maximize the visual impact of contrast enhancement and permit reformatting into other planes or the use of 3D reconstruction techniques.
Timing the scan acquisition to coincide with the appropriate vascular phase of contrast enhancement is key in CE-MRA. In general, venous phase imaging can be obtained at a fixed delay after contrast injection, because venous opacification is related to the distribution of contrast material throughout the circulation. However, for arterial phase imaging, precise timing is required to synchronize image acquisition with the arrival of contrast material. This timing can be accomplished several ways in adults, with automatic bolus tracking techniques/software generally being preferred. This goal can be accomplished in older children who can suspend respiration and have similar cardiovascular physiology to adults. However, in younger children who are unable to cooperate with breath holding and technologist commands, a dynamic postcontrast technique can be used, in which multiple T1-weighted data sets are obtained in rapid succession. This strategy increases the odds that at least 1 of the data sets has a pure arterial phase. The increased speed of acquisition/temporal resolution may necessitate either using parallel imaging techniques or a decrease in spatial resolution.
The use of multiple dynamic phase imaging sequences in the pediatric population has been termed time-resolved MRA in several studies and offers an excellent solution to arterial timing issues in children. Terminology overlaps with time-resolved MRA or keyhole MRA in adults, in whom the term typically refers to techniques that highly undersample k-space to increase scan temporal resolution, and subsequently reconstructing the full k-space data using full k-space acquisitions at the beginning or end of the sequence. The result of these techniques is the ability to rapidly sample the changing tissue contrast of a slice/volume during the administration of contrast and maintain spatial resolution by back-filling the spatial k-space data from other time points, because the vascular system does not move substantially during scan acquisition. Although this latter adult technique may be of benefit in the pediatric population, it has yet to gain major acceptance for pediatric body MRA applications.
Future directions
A blood pool contrast agent, gadofosveset trisodium (Lantheus Medical Imaging, North Billerica, MA), has recently been introduced in the United States for vascular imaging. Gadofosveset reversibly binds to serum albumin, leading to an elongated plasma half-life, which results in a blood pool phase of vascular imaging with a long temporal window (up to 60 minutes). In addition, the contrast agent shows substantially greater relaxivity at 1.5 T than traditional contrast agents, resulting in very high signal intensity and contrast, even at reduced dosing. The approved FDA indication for this contrast agent is evaluation of aortoiliac atherosclerotic disease. However, the pharmacokinetics of this contrast agent make it an excellent option for high-quality venous imaging as well. This use of gadofosveset has only recently been reported in children, including for thoracic aortic imaging and high-resolution MR venography. Future applications of this novel contrast agent in the pediatric population seem likely, particularly as the scope of pediatric endovascular therapeutic techniques continues to increase.
Abdominal and pelvic arterial systems
Normal and Variant Anatomy
The abdominal and pelvic arterial vasculature comprises primarily the abdominal aorta and its visceral branches and the left and right common iliac arteries and their branches. The anterior branches of the abdominal aorta include the celiac, superior mesenteric, and inferior mesenteric arteries, which comprise the principal blood supply of the viscera. There is a large amount of anatomic variation amongst these 3 vessels. The gonadal and phrenic arteries also arise anteriorly.
Laterally, the renal arteries and middle adrenal arteries come off the aorta on either side. The abdominal aorta is clinically divided into suprarenal and infrarenal segments. The renal arteries are typically seen coming off the aorta at the approximate level of L2. However, variant anatomy of renal arteries is present in up to 30% of the population, with many having multiple renal artery on one or both sides.
Posteriorly, the lumbar arteries come off on either side of the aorta for each lumbar vertebral level. The middle sacral artery also arises posteriorly at the aortoiliac bifurcation. The aorta typically bifurcates at the L4 or L5 level into the right and left common iliac arteries, which in turn bifurcate into internal and external iliac arteries. The internal iliac artery further subdivides into an anterior and posterior division, whereas the external iliac artery continues on to become the common femoral artery and provides the primary blood supply for the lower extremity. The common femoral artery serves as the most common site of arterial access for invasive procedures, which can lead to arterial injury such as dissection, pseudoaneurysm formation, or occlusion ( Fig. 1 ).
A persistent sciatic artery is a congenital variation occurring in a small percentage of the population (0.025%–0.04%), in which an embryonic lower limb axial artery (sciatic artery) fails to regress and persists as an elongation of the internal iliac artery ( Fig. 2 ). The sciatic artery normally provides the major blood flow to the lower extremity during development but regresses around the sixth week of gestation into portions of the gluteal and popliteal arteries as well as the tibioperoneal trunk. At the same time that this regression is occurring, the external iliac artery and superficial femoral artery develop from a branch of the fifth lumbar artery. Persistent sciatic artery is classified as complete or incomplete depending on the level of development of the external iliac and superficial femoral arteries. There is a slight male predominance, and although it can be seen bilaterally, it is most commonly noted on the right. Persistent sciatic arteries are prone to atheromatous degeneration, aneurysm formation with or without thrombosis or distal embolization, and local compression.
Abdominal aortic narrowing
Abdominal aortic narrowing, particularly suprarenal, typically manifests with hypertension and weakened or absent femoral pulses. This narrowing can be caused by a wide variety of diseases, such as congenital disorders, infectious or inflammatory conditions, and iatrogenic causes. Some investigators group all of these conditions together and commonly refer to them as the middle aortic syndrome. Other investigators recognize a separate specific entity called midaortic dysplastic syndrome, in cases in which suprarenal abdominal narrowing is present, but no clear underlying cause or known associated syndrome can be identified. Although the cause of midaortic dysplastic syndrome is unknown, it is believed to be a congenital arterial dysplasia, and it usually manifests in the second decade of life.
Hypertension occurs as a result of suprarenal aortic narrowing or direct narrowing of the renal arteries themselves, because disease processes that narrow the aorta frequently involve branch vessels as well. The affected kidney perceives a relative hypotension secondary to the upstream stenosis, causing an increase in renin production, resulting in systemic hypertension. In general, between 5% and 10% of all childhood hypertension is attributable to a vascular or renin-mediated mechanism.
The MR appearance of many of these conditions is similar, with segmental ( Fig. 3 ) or focal ( Fig. 4 ) narrowing of the abdominal aorta and its branches ( Fig. 5 ). Entities in which the arterial vasculature is narrowed secondary to extrinsic compression rather than intrinsic disease can be differentiated by MR identification of the extrinsic masses, such as in retroperitoneal neurofibromas in neurofibromatosis type 1. In instances in which aortic narrowing is secondary to an existing systemic condition, the clinical history or laboratory values may aid in differentiation. This information is also particularly helpful in cases in which a syndrome is present, such as in Williams syndrome ( Fig. 6 ) or tuberous sclerosis.
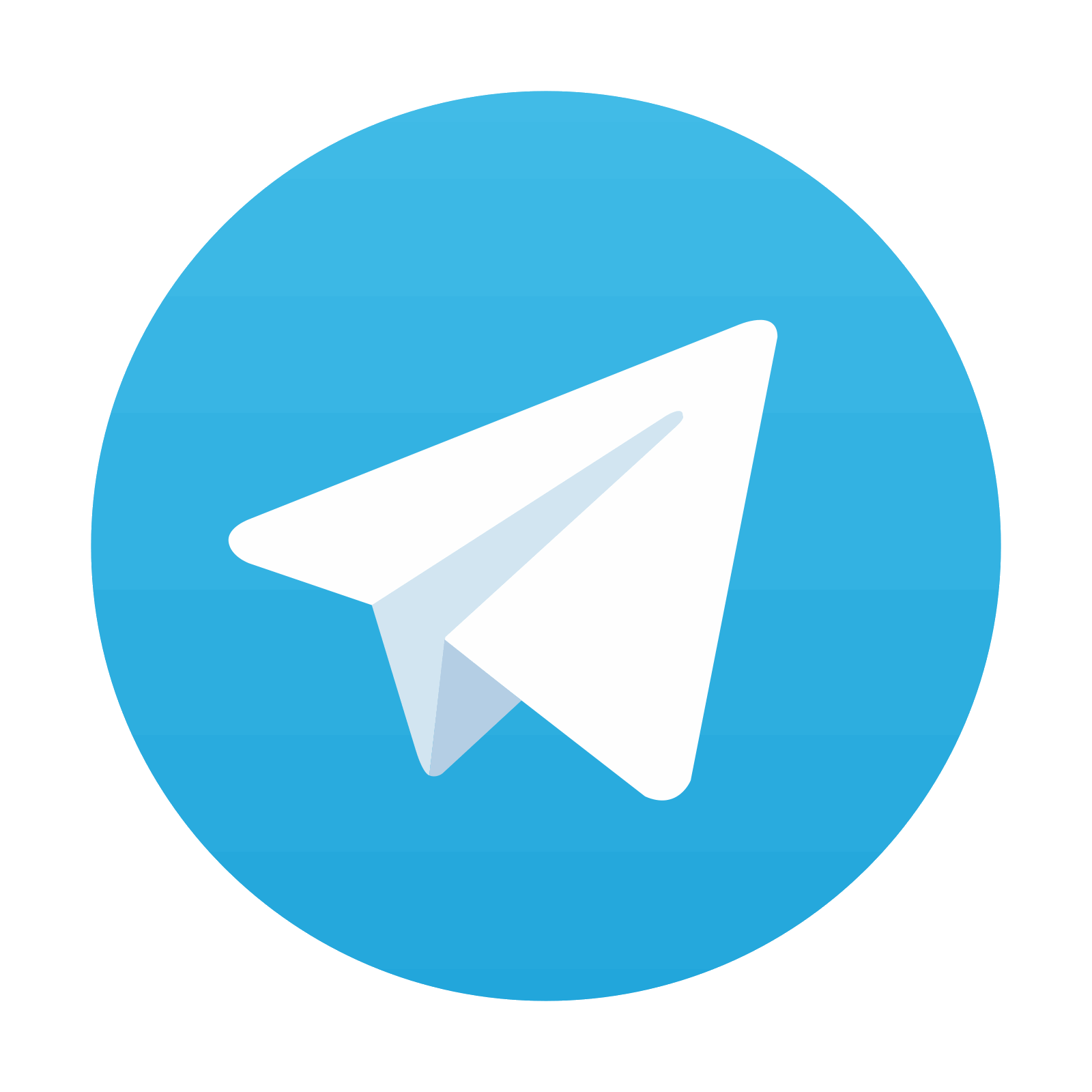
Stay updated, free articles. Join our Telegram channel
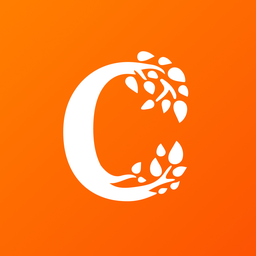
Full access? Get Clinical Tree
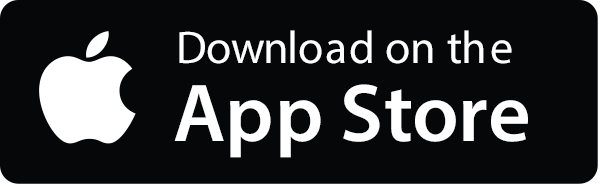
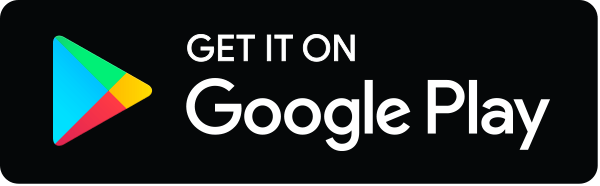
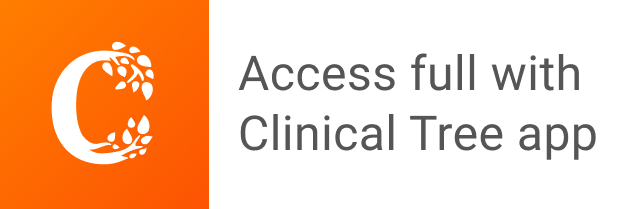