Simultaneous PET-MR imaging improves deficiencies in PET images. The primary areas in which magnetic resonance (MR) has been applied to guide PET results are in correction for patient motion and in improving the effects of PET resolution and partial volume averaging. MR-guided motion correction of PET has been applied to respiratory, cardiac, and gross body movements and shown to improve lesion detectability and contrast. Partial volume correction or resolution improvement of PET governed by MR imaging anatomic information improves visualization of structures and quantitative accuracy. Evaluation in clinical applications is needed to determine their true impacts.
Key points
- •
Combined PET-MR imaging scanners benefit from simultaneity and inherent alignment between the 2 modalities, advantages that are exploited in magnetic resonance (MR)-guided motion correction and MR-guided resolution improvement of PET.
- •
Motion-correction methods involve a coordinated set of MR acquisitions, PET acquisitions, and postprocessing techniques to adjust the PET images for the effects of respiratory, cardiac, or gross body motion occurring during the acquisition.
- •
Motion correction has been shown to improve lesion contrast and detectability in controlled studies but most methods are complex and demanding on scanner resources; their impact on clinical applications remains to be evaluated.
- •
Resolution improvement methods, some of which are referred to as partial volume correction methods, attempt to provide regional or voxelwise PET uptake estimates that are closer to truth by incorporating anatomic information derived from MR imaging.
Introduction
Simultaneous imaging with PET and MR imaging offers the potential for never-before-possible methods of combining and synthesizing information from two complementary imaging modalities. The full potential of this marriage has yet to be explored but recent research offers some glimpses into the clinical utility of proposed techniques. It has long been feasible to acquire PET and MR images on separate scanners, and a significant segment of the research record documents approaches to use the 2 together in clinical applications. The availability of new combined PET-MR imaging scanners, however, calls for a new look at the topic with consideration for the unique capabilities of these devices.
Three principal advantages accrue from the combination of PET and MR imaging into a single scanning device. The first is simultaneity, the ability to view the field of view with both diagnostic modalities at the same time ( Fig. 1 ). Simultaneity is among the most unique aspects of some, though not all, PET-MR imaging scanners because no other combined human-scale imaging system offers this capability. The second principal advantage is alignment, the existence of a common physical geometric reference for both images without a need for additional registration steps. The third principal advantage is the opportunity for reduced time and throughput in scanning when both PET and MR imaging are called for. Although the throughput advantage may be a real consideration in some clinics, and it may contribute to improved alignment by reducing overall imaging time, it has not been shown to appreciably affect the diagnostic quality of the images produced. As a result, this article focuses on reviewing methods that rely primarily on simultaneity and alignment of the PET and MR images.
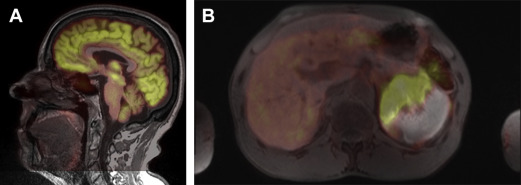
Approaches to technical research in combined PET-MR imaging can be thought of in 3 stages ( Fig. 2 ). The first stage is to address the problems introduced by the substitution of PET-MR imaging for PET-computed tomography (CT), the clinical standard. This stage generally encompasses issues of attenuation mapping and field-of-view mismatch.
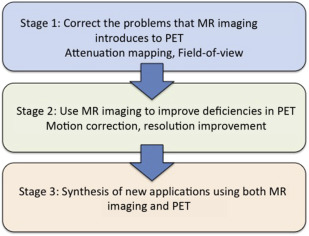
This article will focus on the second stage, encompassing approaches to use MR imaging to address limitations of PET imaging. Most of these methods proposed to date rely on the premise that PET imaging is generally of poorer quality than MR imaging, due largely to physical limitations on spatial resolution, temporal resolution, and noise performance. As a result, it is usually accepted that the MR image can be used to improve the limitations of the PET image and not the other way around. The third stage, still relatively unexplored, is the synthesis of information from both PET and MR imaging to achieve a new goal neither can do alone.
The methods reviewed are classified into 2 primary categories: motion correction, which is the use of MR imaging to provide temporally varying anatomic information used to correct the PET image for motion blurring, and resolution improvement, which is the use of the better spatial resolution and anatomic contrast of MR imaging to improve the inherent spatial resolution of PET.
Introduction
Simultaneous imaging with PET and MR imaging offers the potential for never-before-possible methods of combining and synthesizing information from two complementary imaging modalities. The full potential of this marriage has yet to be explored but recent research offers some glimpses into the clinical utility of proposed techniques. It has long been feasible to acquire PET and MR images on separate scanners, and a significant segment of the research record documents approaches to use the 2 together in clinical applications. The availability of new combined PET-MR imaging scanners, however, calls for a new look at the topic with consideration for the unique capabilities of these devices.
Three principal advantages accrue from the combination of PET and MR imaging into a single scanning device. The first is simultaneity, the ability to view the field of view with both diagnostic modalities at the same time ( Fig. 1 ). Simultaneity is among the most unique aspects of some, though not all, PET-MR imaging scanners because no other combined human-scale imaging system offers this capability. The second principal advantage is alignment, the existence of a common physical geometric reference for both images without a need for additional registration steps. The third principal advantage is the opportunity for reduced time and throughput in scanning when both PET and MR imaging are called for. Although the throughput advantage may be a real consideration in some clinics, and it may contribute to improved alignment by reducing overall imaging time, it has not been shown to appreciably affect the diagnostic quality of the images produced. As a result, this article focuses on reviewing methods that rely primarily on simultaneity and alignment of the PET and MR images.
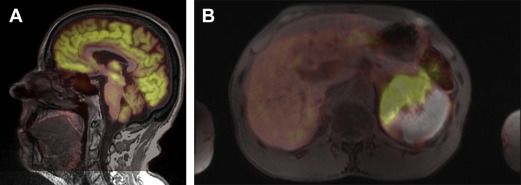
Approaches to technical research in combined PET-MR imaging can be thought of in 3 stages ( Fig. 2 ). The first stage is to address the problems introduced by the substitution of PET-MR imaging for PET-computed tomography (CT), the clinical standard. This stage generally encompasses issues of attenuation mapping and field-of-view mismatch.
This article will focus on the second stage, encompassing approaches to use MR imaging to address limitations of PET imaging. Most of these methods proposed to date rely on the premise that PET imaging is generally of poorer quality than MR imaging, due largely to physical limitations on spatial resolution, temporal resolution, and noise performance. As a result, it is usually accepted that the MR image can be used to improve the limitations of the PET image and not the other way around. The third stage, still relatively unexplored, is the synthesis of information from both PET and MR imaging to achieve a new goal neither can do alone.
The methods reviewed are classified into 2 primary categories: motion correction, which is the use of MR imaging to provide temporally varying anatomic information used to correct the PET image for motion blurring, and resolution improvement, which is the use of the better spatial resolution and anatomic contrast of MR imaging to improve the inherent spatial resolution of PET.
Magnetic resonance–based motion correction of PET images
PET image quality principally depends on the number of photons acquired from the field of view during the scan period. As a result, PET acquisitions generally extend over several minutes per position, with whole-body scans requiring 20 to 30 minutes. Because breath-holding is not feasible, PET is highly susceptible to patient motion, both involuntary and voluntary, resulting in motion blur of the target. Such blurring presents an unrealistic representation of the true PET tracer distribution, increases the apparent size of the target, and reduces the apparent uptake of the target. Simultaneous PET-MR imaging offers the ability to acquire MR images of the subject’s dynamically changing anatomy at the same time as list-mode PET acquisition, in which each photon pair is logged as to its time of acquisition. As a result, magnetic resonance (MR)-driven motion-correction of PET infers from MR imaging how the body position changes during the PET acquisition and then retroactively corrects the PET to reduce motion blurring.
MR imaging–based motion correction techniques are complex and incorporate several coordinated procedures ( Fig. 3 ). The various methods have the following subtasks in common:
- 1.
Motion-state tracking: acquisition of surrogate signals or images during the PET acquisition to infer body pose as a function of time. In conventional PET or PET-CT, this has been done through gating signals acquired from a sensor or detected from the PET data itself through retrospective processing. Combined PET-MR imaging offers the option of determining the motion state from simultaneously acquired fast MR images and many techniques have exploited this (see later discussion).
- 2.
PET framing: organization of the PET data into discrete temporal subunits, each of which comprises a given fixed or nearly fixed body pose. This component is referred to as framing or binning, and is generally derived from the synchronization of the tracking data (component 1) with list-mode acquisition of PET data.
- 3.
Motion modeling: representation of the 3-dimensional (3D) movement of the body within the field-of-view. In combined PET-MR imaging, a patient-specific motion model is often derived from dynamic MR imaging of the subject, before, after, or even during the PET acquisition. The soft-tissue contrast of MR imaging makes it ideal for representing movement of individual organs. Models may be rigid (most often applied to the head) or deformable (often in the thorax). Many different MR strategies have been applied to this component.
- 4.
Tracking-to-model correlation: a methodology for relating the real-time tracking data (component 1) to the 3D motion model (component 3). This component provides an estimate of the body pose for each frame of the PET data, which is used in the final motion-correction step.
- 5.
PET correction: the process of assembling the framed PET data (component 2) into a single motion-corrected PET image guided by body pose information (component 4). This procedure may be done in a postreconstruction format in which PET images are reconstructed for each frame, and then each warped to a common reference body pose and summed. Alternately, many approaches apply a within-reconstruction approach, wherein the body-pose models are incorporated into the forward model of a single PET reconstruction process.
The proposed methods differ primarily in the choices made for the 5 components previously listed. The type of motion, cyclic or noncyclic ( Fig. 4 ), and the target region often determine aspects of those choices (see later discussion).
Cyclic Motion: Respiratory
Respiratory cyclic motion primarily affects targets in the lungs, upper abdominal organs, and the heart. Movement of the diaphragm in normal free breathing is generally measured to be 1 to 2 cm, whereas PET resolution of whole-body systems is on the order of 4 to 5 mm. As a result, oncology targets in the lung, liver, and pancreas may suffer decreased uptake estimates and increased apparent size due to motion blurring ( Fig. 5 ). Studies have estimated these effects through simulations, physical phantoms, and human subjects, and found that the degradations due to motion may be from 25% to 50% in terms of standard uptake value.

Respiratory motion has also been shown to affect the alignment of PET and MR images in simultaneous PET-MR imaging. Because PET is acquired in free breathing and some MR imaging sequences are typically acquired with breath holding, misalignment of simultaneous PET-MR images can be as much as 1 to 4 cm for upper abdominal organs when fast-breath-hold MR imaging sequences are used. This problem may be ameliorated with free-breathing MR imaging sequences that more closely match the anatomy of the PET acquisition.
Another important effect of respiratory motion in PET-MR imaging is the mismatch between the MR-derived attenuation map, which is often acquired with breath holding, and the attenuation experienced during free-breathing. This leads to the oft-cited banana artifact of overestimation of PET activity around the diaphragm (see Fig. 5 B) and inaccuracies in other areas. The errors due to mismatched attenuation correction have been estimated to be from 20% to 30%. Most methods for respiratory motion correction use the body pose information from component 4 to warp the attenuation map as well as the PET space.
For respiratory motion, motion-state tracking may be performed either with external sensor signals, MR navigator acquisitions (fast limited-field-of-view sequences designed to track the diaphragm position), or with analysis of MR images acquired during the PET acquisition. External sensors do not interfere with other imaging procedures but robustness and accuracy may depend on the sensing technology and how it is applied. A good summary of the technologies available for sensing is found elsewhere. The image-based navigator approach has been found to be effective, improving PET contrast and signal-to-noise ratio by 20% to 25% and reducing apparent PET full-width half-maximum resolution by 20%. Interspersing the navigator sequence into other MR acquisitions may be problematic when the navigator suppresses MR signal in desired areas, leading to inefficiencies in acquisition of multiple MR sequences during the session. Similar issues apply to the use of dedicated tracking images acquired at regular intervals during the PET acquisition. An ideal method would be free of sensors and allow for any MR sequences to be run during the PET acquisition. Recently, and somewhat surprisingly, investigators have examined derivation of the tracking signal directly from the PET data, thus removing the MR from the demands of tracking entirely, although these methods still use the MR imaging to derive the patient-specific model component. Such methods are intended to create a more clinically practical approach to motion tracking, and were shown to reduce PET lesion displacement to within 4 mm 2 and to improve standardized uptake value (SUV) 18% to 25%, slightly less effective than fully MR-based motion correction, which had SUV improvement of 23% to 35%.
Motion modeling for respiratory motion is necessarily deformable, therefore requiring a more complex spatial model with many degrees of freedom to represent the space of allowable transformations. The modeling method and tracking-to-model correlation are chosen to work together effectively. A straightforward method is to create a discrete sequence of 3D representations of the body pose at different points in the respiratory cycle, and then to frame the PET data into those discrete poses by establishing discrete thresholds of the tracking signal. More complex models seek to represent the motion space as a continuum of body poses, allowing for realistic interpolation of poses between discrete frames. These approaches require a means of estimating the interpolation parameters from the tracking signal, which has been achieved using regression or machine-learning methods. Both discrete and continuous models depend on the quality of the MR-based modeling images, and the number of frames (usually 6–8 are sufficient) acquired.
A variety of MR imaging methods have been used to acquire the 4-dimensional datasets needed for the modeling. Sensor-based, respiratory-gated MR imaging acquired before or after PET is a standard method available but it demands significant additional acquisition time. Tagged MR imaging acquisition, in which an excitation pattern superimposed on the tissue is tracked as it deforms, offers the ability to track motion to high spatial resolution within tissues that normally respond uniformly in standard gated imaging but also incurs a significant time cost. Some strategies try to achieve improved temporal resolution by using fast 2-dimensional MR imaging during the PET acquisition to infer the 3D body pose from a separately acquired model. Finally, methods using radial MR imaging acquisition during the PET acquisition offer efficiency in (1) not requiring additional MR scans before or after the PET acquisition and (2) performing double duty as both the source of respiratory tracking self-gating data (component 1, via the changes in the zero-frequency k-space component common to each radial line) and of motion modeling images (component 3, reconstructed from multiple gated radial lines).
The respiratory-motion correction of PET images has been examined with both postreconstruction and within-reconstruction techniques. Postreconstruction methods are simpler to implement but may be less accurate overall than within-reconstruction methods. One comparison showed that there was relatively little difference in performance, whereas another showed improved spatial resolution at the cost of poorer noise rejection for within-reconstruction methods. Overall, it is inconclusive whether either offers a better overall tradeoff between noise and spatiotemporal resolution because there are no clear accepted standards for evaluating these techniques.
Cyclic Motion: Cardiac
Motion correction for the heart in PET-MR imaging has been studied somewhat less than respiratory motion, probably owing to the relative clinical importance of oncology PET applications versus cardiac PET applications. Nevertheless, most of the same principles apply and the same approaches can, in many cases, be used. The complex twisting motion of the myocardium affects estimation of the severity and extent of myocardial lesions, and techniques for PET-only motion correction have existed long before combined PET-MR imaging.
Motion-state tracking is achieved rather robustly with the electrocardiogram (ECG) R-wave trigger, and this is a common feature in PET and MR imaging systems. Framing of the PET data is then achieved with synchronization of the ECG trigger signal and the PET list-mode acquisition.
The unique contribution of combined PET-MR imaging for cardiac motion-correction lies in the use of MR imaging to model the motion of the heart. Only tagged-MR imaging methods have been studied for this purpose because they offer the ability to track within-myocardium deformations such as the rotational twisting. Although tagging methods have traditionally been time-consuming, recent work has addressed this with accelerated acquisition methods that may improve their practicability. Discrete modeling of cardiac cycle states has generally been considered sufficient to provide adequate correction.
Because the heart is affected both by its own beating motion and by the respiratory motion of the diaphragm, some efforts have been made to combine both cardiac and respiratory motion models into 5-dimensional or dual-gated approaches. Such approaches are both technically challenging and computationally demanding but they may offer very clear images of the myocardium or coronary arteries ( Fig. 6 ) and may be especially important in quantitative cardiac PET for new radiotracers in the future.
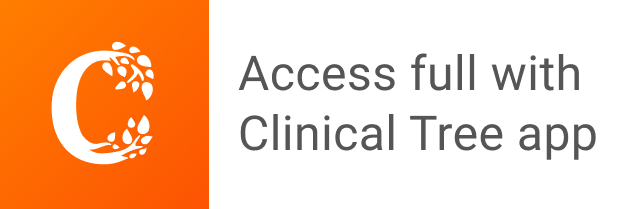