PET/MR imaging benefits neurologic clinical care and research by providing spatially and temporally matched anatomic MR imaging, advanced MR physiologic imaging, and metabolic PET imaging. MR imaging sequences and PET tracers can be modified to target physiology specific to a neurologic disease process, with applications in neurooncology, epilepsy, dementia, cerebrovascular disease, and psychiatric and neurologic research. Simultaneous PET/MR imaging provides efficient acquisition of multiple temporally matched datasets, and opportunities for motion correction and improved anatomic assignment of PET data. Current challenges include optimizing MR imaging-based attenuation correction and necessity for dual expertise in PET and MR imaging.
Key points
- •
Simultaneous PET/MR imaging acquires spatially and temporally matched anatomic MR imaging, MR-based physiologic imaging, and PET metabolic imaging of the brain for clinical care and research.
- •
Combined data obtained may provide a better estimate of tumor extent, better tumor grading, tools therapy optimization, and better differentiation of tumor recurrence from radiation necrosis.
- •
MR imaging is the first-line imaging modality potential surgical intervention for medically refractory epilepsy. PET may support a suspected epileptogenic focus.
- •
PET analysis provides biomarkers of the pathologic processes involved in Alzheimer’s disease, aiding in the prediction of progression and confirmation of Alzheimer’s disease in clinical trials.
Introduction
PET and MR imaging are established imaging modalities for the study of disorders of the central nervous system, with applications in neurooncology, epilepsy, dementia syndromes, cerebrovascular disease, neurologic disease, and psychiatric disorders. The 2 modalities offer complimentary information, with conventional MR imaging providing structural imaging of the brain with high spatial resolution and high tissue contrast, and PET providing physiologic information about brain metabolism. In the last 2 decades, advanced MR imaging techniques, including perfusion-weighted imaging (PWI), functional MR imaging (fMR imaging), MR spectroscopy (MRS), and diffusion-weighted imaging (DWI), and several PET imaging agents targeting numerous metabolic pathways in the brain have expanded the tools available for studying the nervous system in the normal state and in various diseased states. Fusion of MR imaging and PET images obtained at different time points is commonly performed for clinical and research purposes, but the possibility that the physiologic processes studied by PET and by advanced MR techniques may differ slightly during the 2 different imaging sessions limits the ability to cross-validate emerging imaging techniques and may leave open questions about how the findings on the 2 imaging modalities relate to each other because there may be changes in the disease between imaging sessions. Brain imaging was one of the first applications of simultaneous PET/MR imaging and the development of commercial simultaneous PET/MR imaging units allows for true multiparametric analysis of the brain using the 2 modalities. This review focuses on the applications suited to hybrid PET/MR imaging scanners that acquire PET and MR imaging simultaneously.
Introduction
PET and MR imaging are established imaging modalities for the study of disorders of the central nervous system, with applications in neurooncology, epilepsy, dementia syndromes, cerebrovascular disease, neurologic disease, and psychiatric disorders. The 2 modalities offer complimentary information, with conventional MR imaging providing structural imaging of the brain with high spatial resolution and high tissue contrast, and PET providing physiologic information about brain metabolism. In the last 2 decades, advanced MR imaging techniques, including perfusion-weighted imaging (PWI), functional MR imaging (fMR imaging), MR spectroscopy (MRS), and diffusion-weighted imaging (DWI), and several PET imaging agents targeting numerous metabolic pathways in the brain have expanded the tools available for studying the nervous system in the normal state and in various diseased states. Fusion of MR imaging and PET images obtained at different time points is commonly performed for clinical and research purposes, but the possibility that the physiologic processes studied by PET and by advanced MR techniques may differ slightly during the 2 different imaging sessions limits the ability to cross-validate emerging imaging techniques and may leave open questions about how the findings on the 2 imaging modalities relate to each other because there may be changes in the disease between imaging sessions. Brain imaging was one of the first applications of simultaneous PET/MR imaging and the development of commercial simultaneous PET/MR imaging units allows for true multiparametric analysis of the brain using the 2 modalities. This review focuses on the applications suited to hybrid PET/MR imaging scanners that acquire PET and MR imaging simultaneously.
PET/MR imaging protocols
Simultaneous PET/MR imaging of the central nervous system is most beneficial when conventional MR imaging sequences are optimized for depicting the relevant structural anatomy, advanced MR imaging sequences are used to study the relevant physiologic state of the brain, and the PET radiotracer and image acquisition are chosen to best depict the metabolic state of the brain. MR imaging protocols targeted to specific clinical or research questions, such as oncology, epilepsy, and dementia imaging, may be replicated easily on the simultaneous PET/MR imaging unit, allowing for straightforward comparison to MR imaging examinations performed without simultaneous PET. Similarly, PET imaging protocols are easily replicated with results of PET brain imaging acquired on PET/MR imaging units with MR imaging attenuation correction performing similarly to PET brain imaging acquired with conventional CT attenuation correction. Initial studies showed good performance of hybrid PET/MR imaging compared with PET/CT.
Most commonly, PET/MR brain imaging is performed alone, without additional body imaging, although PET/MR imaging may be used to study the remainder of the body, particularly for the workup of metastatic disease to the brain from a source outside the central nervous system. The minimum MR imaging sequences necessarily include the MR imaging attenuation correction sequences, which depend on the MR imaging attenuation correction technique used by the manufacturer and clinical or research site, and basic anatomic brain imaging sequences as dictated by the local site ( Box 1 ). Commercial software for viewing simultaneous PET/MR imaging studies can fuse the PET images with any acquired conventional MR imaging sequence, including T1-weighted imaging (T1WI), T2-weighted imaging, DWI, susceptibility-weighted imaging, and contrast-enhanced T1WI. At our institution, we optimize imaging for epilepsy by acquiring high spatial resolution imaging of the brain using T1WI, fluid-attenuated inversion recovery, and T2-weighted imaging sequences in the coronal plane, for tumors by acquiring high spatial resolution postcontrast T1WI and fluid-attenuated inversion recovery sequences, and for dementia by acquiring high spatial resolution magnetization prepared rapid acquisition gradient echo sequences for quantitative volumetric analysis and PWI for assessment of cerebrovascular disease. Advanced imaging sequences including PWI, MRS, DTI, and fMR imaging may be obtained as well with results comparable with images acquired on a dedicated MR imaging device.
MR Acquisition
- •
Dixon sequence for PET attenuation correction (modify if using an alternative method of attenuation correction).
- •
Conventional brain MR imaging sequences.
- •
For tumor evaluation consider including PWI, MRS, fMR imaging, and DTI.
- •
For epilepsy evaluation consider including coronal plane T1-weighted imaging, T2-weighted imaging, fluid-attenuated inversion recovery imaging.
- •
For dementia evaluation consider including MPRAGE for quantitative volumetric analysis and PWI.
PET Acquisition
- •
PET tracer may be injected either before the scan or during the scan depending on the indication.
- •
Simultaneously acquire PET data during MR image acquisition.
- •
Tumor evaluation: 18 F-FDG, amino acid analogs, 18 F-3′-deoxy-3′-fluorothymidine ( 18 F-FLT), 18 F-fluoromisonidazole ( 18 F-MISO).
- •
Epilepsy evaluation: 18 F-FDG.
- •
Dementia evaluation: 18 F-FDG, amyloid-specific tracers, and tau-specific tracers.
Abbreviations : DTI, diffusion tractography; 18 F-FDG, [18F]-L-dihydroxyphenylalanine; fMR imaging, functional MR imaging; MRPAGE, magnetization prepared rapid acquisition gradient echo; MRS, MR spectroscopy; PWI, perfusion-weighted imaging.
Numerous PET imaging agents targeted to specific disease processes may be used with PET/MR. The most well-known is PET radiotracer 2-[fluorine-18]fluoro-2-deoxy- d -glucose ( 18 F-FDG) and it the most commonly used Food and Drug Administration (FDA)-approved radiotracer for clinical tumor imaging, epilepsy imaging, and dementia imaging. It is widely available in areas of the world that routinely perform clinical PET. There are currently 3 FDA-approved 18 F-labeled amyloid-β PET imaging agents including 18 F-florbetaben, 18 F-florbetapir, and 18 F-flutemetamol. Multiple other radiotracers, including those targeting tau protein in Alzheimer disease (AD) research and amino acid metabolites in brain tumor research, are not currently FDA approved. Limited access to cyclotron facilities capable of producing many of these PET tracers limits their use to research facilities.
The portion of the PET/MR examination specific to PET imaging includes the injection and uptake period for the PET tracer, acquisition of the MR images required for PET attenuation correction, and collection of PET data. Brain-only PET imaging may allow for the injection and uptake phase of the PET examination to occur while the targeted MR imaging is being acquired, thus increasing the efficiency of the examination acquisition for both the patient and the PET/MR facility. Some PET studies benefit from the collection of dynamic PET data or PET images at more than 1 time point after injection, which is easily coordinated within the sequence of MR image acquisition because PET data can be collected simultaneously. Long PET acquisition times are also possible, thus increasing total collected counts and signal-to-noise ratio.
PET/MR Imaging Benefits and Challenges
PET/MR imaging offers several advantages over separately acquired conventional PET/CT and MR imaging ( Box 2 ). The practical advantage of simultaneously acquiring PET and MR imaging during a single imaging session eliminates possible delays in scheduling 2 separate examinations; potentially improves patient satisfaction because the patient only needs to travel to the imaging facility and take time away from other daily activities for a single examination rather than 2 examinations; and patients requiring sedation for PET and MR imaging only need to undergo sedation a single time for a shorter total time length. A PET/MR imaging study only uses ionizing radiation for the radiotracer used for the PET imaging, saving the added radiation necessary for attenuation correction and anatomic correlation used in the CT portion of a PET/CT scan, which is beneficial in young patients, pregnant patients, and patients with a potentially long lifespan who may need multiple PET imaging studies during their remaining lifetime. PET/MR imaging may add value by promoting multidisciplinary interpretations with PET and neuroimaging experts. Although commercially available fusion software does a good job of fusing separately acquired PET/CT scans with MR imaging, only simultaneous PET/MR imaging can provide temporally matched information for disease states that may change between the time it takes to acquire 2 separate imaging studies. Patient head motion during PET acquisition may interfere with appropriate attenuation correction or lead to misregistration of the PET data to the MR images. MR imaging-based motion tracking may be used to realign the PET data. The PET spatial resolution obtained from the Siemens mMR Biograph simultaneous PET/MR (Siemens, Erlangen, Germany) has been measured at 4.3 mm, which is greater than typical human cortical thicknesses. Activity in the thin cortical layer may be localized falsely to adjacent areas. The MR imaging component of the scan may be used to create an anatomic mask for gray matter and white matter to improve assignment of PET activity to tissue on the MR image. Quantitative brain PET relies on an input function obtained from arterial sampling to estimate delivery of the tracer to the binding site. PET/MR imaging provides a means for noninvasively measuring the arterial input function from the internal carotid artery, validated against conventional arterial blood sampling and 15 O-H 2 O measurement of cerebral blood flow.
Benefits
- •
Improved efficiency by acquiring PET and MR datasets during a single examination.
- •
MR imaging-based attenuation correction reduces total radiation dose.
- •
Temporally and spatially matched anatomic MR imaging, PWI, DWI, and MRS data with PET metabolic data.
- •
Simultaneous acquisition reduces error in combined interpretation of MR physiologic and PET metabolic data, which may fluctuate across time as disease states and therapies change.
- •
Opportunity for combined interpretations from PET and MR experts that may provide added benefit over separately interpreted PET and MR studies.
- •
Potential for MR imaging-based motion correction for PET.
- •
Potential for MR imaging-based gray–white matter mask to improve localization of PET activity.
- •
Noninvasive MR imaging-based tools for measuring arterial input function for quantitative brain PET.
Challenges
- •
MR attenuation correction techniques require further validation.
- •
Availability of PET/MR imaging scanners for clinical use remains limited to large medical centers.
- •
Two sets of technologists and physicians with expertise in PET and MR imaging, or a single technologist and physician with dual training in PET and MR imaging, are required to acquire and interpret PET/MR imaging studies.
Abbreviations : DWI, diffusion-weighted imaging; MRS, MR spectroscopy; PWI, perfusion-weighted imaging.
PET/MR imaging poses challenges for the PET and MR imaging communities (see Box 2 ). MR imaging-based attenuation correction strategies for the brain, discussed in detail elsewhere, are an ongoing area of research. The most common strategy used for pure MR-based attenuation correction in clinical scanners uses the Dixon MR technique creating an attenuation correction map consisting of tissue, air, fat, and water. This technique does not account for attenuation owing to bone, which is relevant to brain imaging because the brain is surrounded by the skull. Studies are ongoing evaluating the impact of this attenuation correction technique on brain imaging as well as developing alternative methods. Hitz and colleagues reported that the measured uptake of 18 F-FDG in the brains of dementia patients was lower on a PET/MR scanner compared with a PET/CT scanner. This was true for both attenuation-corrected and non–attenuation-corrected data. A new cohort of normal control patients imaged with PET/MR techniques may be necessary for future clinical trials, which may require quantitative PET. There are few physicians with dual expertise in these modalities. The American College of Radiology and the Society of Nuclear Medicine and Molecular Imaging recommend that the MR imaging component and PET component should be interpreted by physicians who meet qualifications standards in the 2 modalities. PET/MR imaging is ideally interpreted as a single study using both PET and MR imaging information to arrive at a final interpretation. At some institutions, this may require joint interpretation by PET and MR imaging experts. Cross-modality training should also be considered in residency and fellowship programs as well as professional societies offering continuing medical education. Similarly, the skill set and knowledge base required for meeting the technical and safety standards for acquiring PET and MR imaging differ, necessitating the crosstraining of PET and MRI technologists in the complimentary modality to safely acquire a PET/MR imaging. The Society of Nuclear Medicine and Molecular Imaging Technologist Section and the Section for Magnetic Resonance Technologists recommend that technologists involved in acquiring PET/MR imaging meet qualification standards for MR imaging and PET individually, which may either require MR imaging and PET technologists, or a single, dually trained technologist with advanced training in the field secondary to their area of expertise.
Applications
Oncology
Contrast-enhanced MR imaging is the first-line imaging study for the diagnosis, preoperative planning, and treatment monitoring of both primary and metastatic brain tumors. Advanced MR imaging techniques including PWI, DWI, MRS, diffusion tractography (DTI), and fMR imaging provide physiologic information about the tumor and adjacent brain, which complements conventional MR imaging in both clinical and research settings.
PET uses radiotracers targeted to different aspects of tumor metabolism: glucose metabolism ( 18 F-FDG), amino acid transport and protein synthesis ( 11 C-MET [ 11 C-methionine], 18 F-FET [ O -(2- 18 F-fluoroethyl)- l -tyrosine], 18 F-DOPA), proliferation rate and DNA synthesis ( 18 F -FLT), and oxygen metabolism and hypoxia ( 18 F-MISO). Although not practical for clinical use owing to the short half-life, 15 O-H 2 O is used to study tumor perfusion. Although PET does not have a primary clinical role in oncologic imaging of the brain, 18 F-FDG PET scanning of the brain is used clinically most commonly to differentiate radiation necrosis from recurrent tumor. The radiotracers targeting other metabolites are promising because of improved signal-to-background noise ratio and specificity for tumor compared with 18 F-FDG. PET scanning has been used in glioma research using multiple tracers beyond 18 F-FDG targeting different aspects of tumor metabolism to study tumor grading, tumor extension, treatment planning, treatment follow-up, and prognosis, as well as to validate physiologic information gained from advanced MR imaging. The combination of MR imaging-based anatomic and physiologic imaging with temporally and spatially matched PET-based molecular imaging allows us to answer questions pertaining to prognosis, plan therapeutic approaches, and assess response to treatment with greater confidence than is possible with either modality alone ( Box 3 ).
Simultaneous PET/MR imaging provides benefit by ensuring that MR imaging physiologic data and PET metabolic data reflect the same biological state, eliminating the chance that change in therapy between separate imaging sessions may cause discordant results.
Glioma Grading
- •
Contrast enhancement, elevated regional cerebral blood volume, lactate peak on MRS, and low apparent diffusion coefficient suggest high-grade glioma.
- •
18 F-FDG, 11 C-MET, 18 F-DOPA, and 18 F-MISO can differentiate high-grade glioma from low-grade glioma.
Tumor Extension
- •
PWI, DTI/DWI, MRS, and amino acid analog PET tracers aid in determining nonenhancing tumor margins.
Treatment Follow-Up
- •
MRS, PWI, DWI, 18 F-FDG PET, and amino acid PET aid in differentiating radiation necrosis and pseudoprogression from recurrent high-grade glioma
Metastatic Disease
- •
Conventional contrast-enhanced MR imaging is primarily used for detection of intracranial metastatic disease and follow-up after treatment.
- •
Whole-body 18 F-FDG PET/MR imaging including dedicated contrast-enhanced brain imaging may be equivalent to PET/CT and brain MR imaging for staging metastatic lung cancer.
Abbreviations : 11 C-MET, 11 C-methylmethionine; DTI, diffusion tractography; 18 F-FDG, 2-[fluorine-18]fluoro-2-deoxy- d -glucose; 18 F-DOPA, [18F]-L-dihydroxyphenylalanine; fMR imaging, functional MR imaging; MRS, MR spectroscopy; PWI, perfusion-weighted imaging.
Tumor grading and differential diagnosis
The differential diagnosis of intracranial masses and initial estimation of tumor grade begins with conventional MR imaging. Contrast enhancement owing to breakdown of the blood–brain barrier is the imaging marker used in MR imaging to differentiate high-grade gliomas (HGG) from low-grade gliomas (LGG), and to detect transformation of an LGG to an HGG. Histopathologic evaluation of brain tumors remains the gold standard for assessing tumor grade because as many as one-third of HGG may not enhance. Additionally, stereotactic biopsy may undersample a tumor, leading to an inappropriately lower histologic grade. PWI, DWI, and MRS provide additional physiologic information and PET scanning provides additional metabolic information to delineate regions of the tumor of highest grade for biopsy planning and to detect transformation of an LGG to an HGG with greater confidence.
PWI interrogates the vascularity of tumor and the surrounding tissue. Within the astrocytoma line of tumors, greater regional cerebral blood volume (rCBV) corresponded with a higher tumor grade. The rCBV aids in differentiating neoplastic from nonneoplastic lesions, provides an estimate of tumor grade, and may be used to direct biopsy toward the portion of the tumor with the potential highest grade. Caution is necessary in using the results because there is variability in techniques for processing and interpreting MR perfusion, which influences results, and the major techniques do not yield the same values when applied to the same tumor. The apparent diffusion coefficient value obtained from DWI corresponds with the high cellularity seen at pathology in HGG. DWI can predict glioma grade, with higher grade tumors having lower apparent diffusion coefficient values. An altered metabolite spectrum with 1 H-MRS suggests tumor grade. 1 H-MRS demonstrates increased choline owing to increased cellular membrane synthesis and reduced N -acetylaspartate owing to neuronal loss in the setting of HGG. Increased lactate suggests tumor hypoxia and lipid indicates necrosis as is seen in HGG histopathology. MRS is technically challenging to acquire in clinical practice with overlap seen between tumors and tumor mimickers.
High-grade tumors have a high rate of glucose metabolism and increased 18 F-FDG uptake. 18 F-FDG uptake correlates with high glioma grade and poorer survival. Newly increased 18 F-FDG uptake within an LGG signals conversion to HGG. Inherent high 18 F-FDG background activity in the brain results in poor delineation of some primary brain tumors and small metastases compared with MR imaging, preventing the routine use of 18 F-FDG in brain tumor imaging. Coregistration of 18 F-FDG PET with MR imaging is critical because 18 F-FDG uptake of tissue must be correctly assigned to white matter, gray matter, or abnormal tissue for proper interpretation. Alternative PET tracers, including 18 F-DOPA, 18 F-MISO, and 11 C-MET, have been studied as well for predicting tumor grade and time to progression, but are not in routine clinical use.
Tumor extension and treatment planning
MR imaging defines the margins of enhancing and nonenhancing primary and metastatic tumors for surgical resection, but there is overlap in the appearance of nonenhancing glioma margins and vasogenic edema on T2-weighted imaging. Advanced MR imaging sequences, including diffusion tensor imaging, MRS, and PWI, and PET imaging with amino acid analogs aid in differentiating tumor margin from edema. PWI, particularly rCBV, and fractional anisotropy derived from diffusion tensor imaging differ between nonenhancing tumor and peritumoral edema. Amino acid analogs are well-suited to this problem because they have high uptake in tumor and low uptake in normal brain, which improves tumor tissue contrast compared with 18 F-FDG. 11 C-Methionine and 18 F-FET PET have been shown to differentiate tumor margin from edema, with 18 F-FET PET shown to be superior to 18 F-FDG PET in determining extent of tumor. 18 F-DOPA also shows promise in ability to delineate tumor margins for surgical resection and radiotherapy planning ( Fig. 1 ). Multiparametric maps including MR imaging and PET sequences may lead to maps of the brain delineating on a voxel-by-voxel basis the expected grade of tumor and delineate the full extent of tumor. Simultaneous PET/MR imaging efficiently combines structural MR imaging with advanced MR data and metabolic PET data for complete pretreatment evaluation of the tumor.
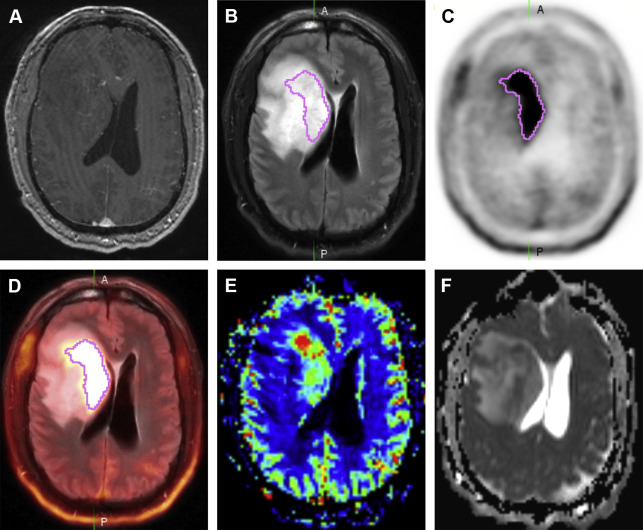
Tractography and fMR imaging play a role in preoperative planning before biopsy or resection of gliomas and metastases by delineating eloquent cortex and the associated fiber tracts. Incorporating fMR imaging and DTI into the preoperative workup facilitates maximal resection with reduced risk of loss of neurologic function as a result surgery. Neuschmelting and colleagues recently reported using PET/MR imaging with 18 F-FET PET, contrast-enhanced MR imaging, tractography, and navigated transcranial magnetic stimulation to map the relationships between enhancing tumor, metabolically apparent tumor, and eloquent cortex and tracts for surgical planning.
Treatment follow-up
Contrast-enhanced MR imaging is the key imaging study for following response to therapy, recurrence, and development of treatment-related changes. Conventional MR imaging is fraught with problems in the posttreatment brain because of difficulties in assessing nonenhancing tumor, after response to antiangiogenic therapy, differentiating pseudoprogression from true early progression, and differentiating recurrent tumor from delayed radiation necrosis. Although structural detail is superior with MR imaging, overlap in the appearance of treatment-related changes and recurrent HGG or metastases supports a role for PET imaging. Simultaneous PET/MR imaging is especially useful because it is a common conundrum in clinical practice that results from MR differ from the results of 18 F-FDG PET. Differences may be owing to changes in therapy initiated between the scans or reflective of complex tumor biology. Simultaneous acquisition of MR physiologic imaging and PET metabolic imaging provides more confidence that all imaging data reflect the same state.
rCBV has been shown to aid in differentiating delayed radiation necrosis from recurrent high-grade tumor. Fink and colleagues showed that multivoxel MRS, specifically choline/creatine and choline/ N -acetylaspartate ratios, and rCBV ratios had equivalent performance in differentiating radiation necrosis from recurrent HGG. Multiparametric maps incorporating apparent diffusion coefficient, rCBV have been shown to correlate with response to treatment. Prat and colleagues showed that MRS and PWI reached 100% positive and negative predictive values for detecting the presence of HGG in treated patients, compared with a positive predictive value of 75% and negative predictive value of 61.1% for 18 F-FDG PET.
One of the earliest applications of 18 F-FDG PET in the brain was differentiating radiation necrosis from recurrent tumor ( Fig. 2 ). 18 F-FLT, 18 F-DOPA, 18 F-FLT, and 11 C-MET with their more favorable tumor-to-background activity characteristics may perform better than 18 F-FDG. In a metaanalysis published in 2013, Nihashi and colleagues reported that 11 C-MET had a summary sensitivity of 0.70 (95% confidence interval [CI], 0.50–0.84) and specificity of 0.93 (95% CI, 0.44–1.0) across 7 studies for detecting recurrence of HGG after treatment compared with a summary sensitivity of 0.77 (95% CI, 0.66–0.85) and specificity of 0.78 (95% CI, 0.54–0.91) across 16 studies for 18 F-FDG PET. The mean lesion-to-normal tissue ratio of 11 C-MET uptake was a good tool for differentiating tumor recurrence from radiation necrosis for both gliomas and metastatic disease. Two studies found that 18 F-DOPA was more accurate than rCBV in detecting recurrent brain metastases from radiation necrosis. Although the body of evidence comparing PET with other imaging modalities is increasing, the body of knowledge remains small.
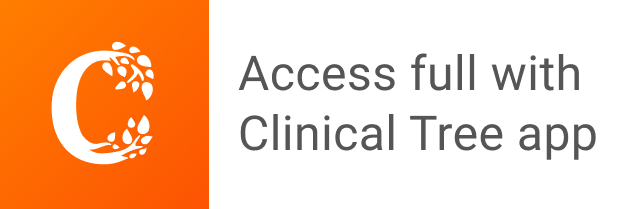