The use of clinical imaging modalities for the guidance of targeted drug delivery systems, known as image-guided drug delivery (IGDD), has emerged as a promising strategy for enhancing antitumor efficacy. MR imaging is particularly well suited for IGDD applications because of its ability to acquire images and quantitative measurements with high spatiotemporal resolution. The goal of IGDD strategies is to improve treatment outcomes by facilitating planning, real-time guidance, and personalization of pharmacologic interventions. This article reviews basic principles of targeted drug delivery and highlights the current status, emerging applications, and future paradigms of MR-guided drug delivery.
Key points
- •
MR imaging can enable planning, monitoring, real-time control, and posttherapy assessment of tumor-targeted drug delivery.
- •
Use of MR imaging to guide the combination of hyperthermia and thermosensitive drug delivery systems constitutes an effective approach for enhancing drug delivery to tumors.
- •
MR-guided high intensity focused ultrasound (MR-HIFU) is a particularly promising technique for improving delivery of systemically administered therapies and potential modulation of the tumor microenvironment.
Advanced drug delivery systems
Targeted drug delivery, whereby therapeutic agents are transported from the site of administration specifically to diseased tissues, remains a “holy grail” of pharmaceutical research. This concept has significant potential in oncology, since side effects from chemotherapeutic drugs with narrow therapeutic windows (the range between effective and toxic doses) can limit the dose and compromise the efficacy of treatment. Recent progress in pharmaceutical nanotechnology has led to the development of a variety of advanced drug delivery systems (DDS) with the capacity to transport small-molecule drugs to tumors, resulting in reduced systemic toxicity and improved treatment outcomes.
In general, DDS-based drug formulations possess several advantages over their conventional counterparts, including (1) enhanced tumor targeting, (2) extended systemic circulation, and (3) controlled drug release. Careful design of DDS can exploit these characteristics to dramatically increase the safety margin of cytotoxic drugs with traditionally narrow therapeutic windows. Examples of DDS used for this purpose include polymeric micelles, liposomes, polymer-drug conjugates, and antibody-targeted therapies ( Fig. 1 ). To date, liposomes have achieved significant success, with several formulations receiving clinical approval and many others, including temperature-sensitive liposomes (TSLs), are in clinical trials.
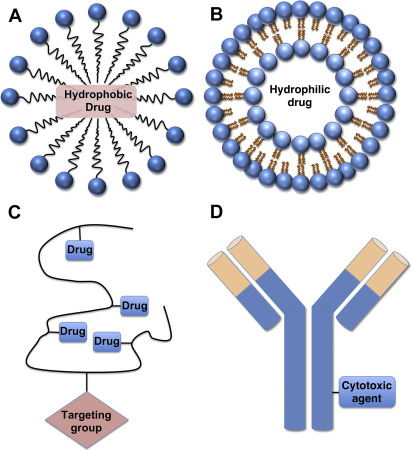
DDS may be “passively” targeted by exploiting features of the tumor microenvironment to enhance drug accumulation, and/or “actively” targeted by binding of DDS to cancer cells or endothelium via specific chemical affinity. Tumor accumulation via passive targeting is achieved because of the characteristically hyperporous blood vessels of neoplasms (resulting in increased extravasation of macromolecules and nanoparticles) and dysfunctional lymphatic drainage, a phenomenon referred to as the enhanced permeability and retention (EPR) effect. Active tumor targeting exploits the specific molecular affinity of bioactive ligands, such as peptides and antibodies, for cellular receptors preferentially expressed in malignant tissues to enhance tumor localization, retention, and cellular uptake.
Barriers to Effective Drug Delivery
Following successful tumor targeting, stable DDS tend to be confined to perivascular tissues because of their limited penetration into the tumor interstitium. If the drug remains bound to or encapsulated by the carrier, concentrations deep within the interstitium and in regions of low vascular density or high interstitial pressure will be limited. Recent efforts to improve drug delivery have focused on increasing both the amount and bioavailability of drugs delivered to a tumor by incorporating external release stimuli into the drug delivery paradigm. Drug release may be triggered by endogenous stimuli such as tissue pH and redox reactions associated with the microenvironment of tumors, or by application of exogenous triggers such as alternating magnetic fields, heat, and light. Unlike endogenous stimuli, the spatiotemporal application of exogenous triggers can be controlled, providing a means for modulating drug release.
Insufficient dosing of tumors during chemotherapy resulting from poor intratumoral drug distribution and penetration is regarded as a major limitation of intravenous drug delivery. Many factors present barriers to drug delivery at the intratumoral level, including inefficient angiogenic vessels, the spatial heterogeneity of the tumor vasculature network, high cellular and stromal density, and elevated interstitial fluid pressure, among others. Indeed, numerous studies have demonstrated the limited penetration of both conventional and DDS-based chemotherapy from tumor blood vessels into the interstitium. These regions, deep within the interstitial space, are prone to transport-mediated and hypoxia-mediated drug resistance, and are a cause of tumor recurrence. Identifying and mitigating transport barriers by means of chemical, thermal, or mechanical alteration of the tumor microenvironment, and by enhanced guidance of drug delivery, are active areas of research.
Image-Guided Drug Delivery
In the emerging era of personalized medicine, the ability to provide optimal treatment to the “right patient at the right dose at the right time” is a central tenet. However, new tools are required to facilitate better customization of therapy based on the specific needs of individual patients. Recently, image-guided drug delivery, which leverages clinical imaging modalities for guidance of DDS, has emerged as a viable strategy for enhancement of targeted, personalized drug therapies. In this drug delivery paradigm, imaging may be used to identify target and nontarget anatomy or for screening, planning, monitoring, and postprocedural assessment of treatment outcome ( Fig. 2 ).

MR imaging is particularly well suited for the purpose of image-guided drug delivery because of its ability to acquire images and quantitative measurements with high spatiotemporal resolution during therapy. MR imaging–guided drug delivery may facilitate or augment existing minimally invasive image-guided therapies, with the eventual goals of improving efficacy or expanding the indications beyond existing local and regional paradigms. The remainder of this article reviews the current state of MR imaging–guided drug delivery, with a focus on hyperthermia-mediated drug delivery and potential future applications.
Hyperthermia-Mediated Drug Delivery
Hyperthermia, in the context of drug delivery and thermal therapy, refers to the application of heat resulting in tissue temperatures greater than normal physiologic temperature. In general, hyperthermia can be divided into mild (∼40°–45°C) and ablative (∼50°–100°C) regimes in which bioeffects are governed by a time-temperature Arrhenius continuum (thermal dose). Commonly used methods for generating local hyperthermia include the use of radiofrequency, microwaves, laser, or superficial hot water applicators. Temperature-sensitive DDS can be used as an adjuvant to thermal ablation to reduce the potential for tumor regrowth in regions of sublethal thermal damage such as at the ablation margin. In addition, mild hyperthermia can be used to trigger drug release within heated tissue and may be used just before subablative or ablative temperature elevation, potentially resulting in a “drug depot” effect whereby drug is concentrated in target tissues following local vascular shutdown. Mild hyperthermia and ablation can also be combined to treat large or conjoined tumors, or geographically distributed tumors that might not otherwise have local or regional interventional oncology options (ie, because of size, number, or distribution).
Advanced drug delivery systems
Targeted drug delivery, whereby therapeutic agents are transported from the site of administration specifically to diseased tissues, remains a “holy grail” of pharmaceutical research. This concept has significant potential in oncology, since side effects from chemotherapeutic drugs with narrow therapeutic windows (the range between effective and toxic doses) can limit the dose and compromise the efficacy of treatment. Recent progress in pharmaceutical nanotechnology has led to the development of a variety of advanced drug delivery systems (DDS) with the capacity to transport small-molecule drugs to tumors, resulting in reduced systemic toxicity and improved treatment outcomes.
In general, DDS-based drug formulations possess several advantages over their conventional counterparts, including (1) enhanced tumor targeting, (2) extended systemic circulation, and (3) controlled drug release. Careful design of DDS can exploit these characteristics to dramatically increase the safety margin of cytotoxic drugs with traditionally narrow therapeutic windows. Examples of DDS used for this purpose include polymeric micelles, liposomes, polymer-drug conjugates, and antibody-targeted therapies ( Fig. 1 ). To date, liposomes have achieved significant success, with several formulations receiving clinical approval and many others, including temperature-sensitive liposomes (TSLs), are in clinical trials.
DDS may be “passively” targeted by exploiting features of the tumor microenvironment to enhance drug accumulation, and/or “actively” targeted by binding of DDS to cancer cells or endothelium via specific chemical affinity. Tumor accumulation via passive targeting is achieved because of the characteristically hyperporous blood vessels of neoplasms (resulting in increased extravasation of macromolecules and nanoparticles) and dysfunctional lymphatic drainage, a phenomenon referred to as the enhanced permeability and retention (EPR) effect. Active tumor targeting exploits the specific molecular affinity of bioactive ligands, such as peptides and antibodies, for cellular receptors preferentially expressed in malignant tissues to enhance tumor localization, retention, and cellular uptake.
Barriers to Effective Drug Delivery
Following successful tumor targeting, stable DDS tend to be confined to perivascular tissues because of their limited penetration into the tumor interstitium. If the drug remains bound to or encapsulated by the carrier, concentrations deep within the interstitium and in regions of low vascular density or high interstitial pressure will be limited. Recent efforts to improve drug delivery have focused on increasing both the amount and bioavailability of drugs delivered to a tumor by incorporating external release stimuli into the drug delivery paradigm. Drug release may be triggered by endogenous stimuli such as tissue pH and redox reactions associated with the microenvironment of tumors, or by application of exogenous triggers such as alternating magnetic fields, heat, and light. Unlike endogenous stimuli, the spatiotemporal application of exogenous triggers can be controlled, providing a means for modulating drug release.
Insufficient dosing of tumors during chemotherapy resulting from poor intratumoral drug distribution and penetration is regarded as a major limitation of intravenous drug delivery. Many factors present barriers to drug delivery at the intratumoral level, including inefficient angiogenic vessels, the spatial heterogeneity of the tumor vasculature network, high cellular and stromal density, and elevated interstitial fluid pressure, among others. Indeed, numerous studies have demonstrated the limited penetration of both conventional and DDS-based chemotherapy from tumor blood vessels into the interstitium. These regions, deep within the interstitial space, are prone to transport-mediated and hypoxia-mediated drug resistance, and are a cause of tumor recurrence. Identifying and mitigating transport barriers by means of chemical, thermal, or mechanical alteration of the tumor microenvironment, and by enhanced guidance of drug delivery, are active areas of research.
Image-Guided Drug Delivery
In the emerging era of personalized medicine, the ability to provide optimal treatment to the “right patient at the right dose at the right time” is a central tenet. However, new tools are required to facilitate better customization of therapy based on the specific needs of individual patients. Recently, image-guided drug delivery, which leverages clinical imaging modalities for guidance of DDS, has emerged as a viable strategy for enhancement of targeted, personalized drug therapies. In this drug delivery paradigm, imaging may be used to identify target and nontarget anatomy or for screening, planning, monitoring, and postprocedural assessment of treatment outcome ( Fig. 2 ).
MR imaging is particularly well suited for the purpose of image-guided drug delivery because of its ability to acquire images and quantitative measurements with high spatiotemporal resolution during therapy. MR imaging–guided drug delivery may facilitate or augment existing minimally invasive image-guided therapies, with the eventual goals of improving efficacy or expanding the indications beyond existing local and regional paradigms. The remainder of this article reviews the current state of MR imaging–guided drug delivery, with a focus on hyperthermia-mediated drug delivery and potential future applications.
Hyperthermia-Mediated Drug Delivery
Hyperthermia, in the context of drug delivery and thermal therapy, refers to the application of heat resulting in tissue temperatures greater than normal physiologic temperature. In general, hyperthermia can be divided into mild (∼40°–45°C) and ablative (∼50°–100°C) regimes in which bioeffects are governed by a time-temperature Arrhenius continuum (thermal dose). Commonly used methods for generating local hyperthermia include the use of radiofrequency, microwaves, laser, or superficial hot water applicators. Temperature-sensitive DDS can be used as an adjuvant to thermal ablation to reduce the potential for tumor regrowth in regions of sublethal thermal damage such as at the ablation margin. In addition, mild hyperthermia can be used to trigger drug release within heated tissue and may be used just before subablative or ablative temperature elevation, potentially resulting in a “drug depot” effect whereby drug is concentrated in target tissues following local vascular shutdown. Mild hyperthermia and ablation can also be combined to treat large or conjoined tumors, or geographically distributed tumors that might not otherwise have local or regional interventional oncology options (ie, because of size, number, or distribution).
High-intensity focused ultrasound
High-intensity focused ultrasound (HIFU) represents a noninvasive alternative to more traditional hyperthermia applications by tightly focusing ultrasonic waves from an external transducer to generate localized temperature elevation in target tissues ( Fig. 3 ). The use of HIFU to generate heat in target tissues is particularly advantageous, as it can be focused deep within the body and can achieve both mild and ablative hyperthermia. The interaction of propagating ultrasound waves with tissue can result in thermal and mechanical bioeffects that can enhance extravasation, uptake, or stimulate drug release from temperature-sensitive or pressure-sensitive DDS. Both thermal and mechanical bioeffects can also be used to enhance intracellular drug delivery and tissue permeability. Furthermore, HIFU-mediated bioeffects can be tuned and controlled by adjusting the device power, frequency, duty cycle, timing, and other settings.
The use of MR imaging for image guidance of HIFU therapy, referred to as MR-HIFU, provides high-resolution anatomic imaging, and the ability to monitor HIFU-mediated temperature changes and tissue displacement. To date, MR-HIFU has been primarily used for the ablation of symptomatic uterine leiomyomata. However, it is currently under investigation for several oncologic applications including treatment of benign breast fibroadenomas, malignant breast carcinoma, and prostate cancer, palliative treatment of painful osseous metastases, and ablation of brain tumors. Moreover, MR-HIFU is being investigated as a treatment for neurologic disorders (eg, epilepsy, essential tremor, neuropathic pain, and Parkinson disease), and as an immunomodulator to inhibit local tumor recurrence and metastases following ablation. MR-HIFU has also been shown to improve drug delivery to the brain by modulation of the blood-brain barrier (BBB).
Temperature-Sensitive Liposomes
Developments in ultrasound applicators and delivery techniques and in MR imaging–based temperature monitoring and control methods have made MR-HIFU–mediated mild hyperthermia an intriguing modality for noninvasive hyperthermia therapies in combination with drug delivery. A particularly attractive strategy for facilitating tumor-localized drug delivery consists of applying mild hyperthermia in combination with intravenous administration of TSLs. When heat is applied to the tumor, circulating TSLs in transit through the tumor vasculature rapidly release the drug, resulting in high intravascular drug concentrations that promote extravasation and penetration into the tumor interstitium ( Fig. 4 ).
TSLs optimized for drug release at mild hyperthermic temperatures between 39.5°C and 41°C are known as low-temperature–sensitive liposomes (LTSLs). Various preclinical studies have demonstrated substantial reductions in tumor volume using LTSLs in combination with mild hyperthermia when compared with conventional therapy or nonthermally sensitive liposomes. Recently, an LTSL formulation containing doxorubicin underwent a phase III clinical trial for the treatment of hepatocellular carcinoma in combination with radiofrequency ablation (RFA).
MR-guided drug delivery
MR imaging offers several advantages over other imaging techniques, such as ultrasonography and computed tomography, which make it ideal for guidance of drug delivery. First, variation between tissue-specific MR parameters enables excellent contrast between soft tissues, and between normal and abnormal morphology/pathology, enhancing precision of the treatment plan. However, MR imaging is devoid of ionizing radiation, which is of particular importance because repeated imaging is usually necessary to monitor and guide drug delivery and to assess tumor progression. Furthermore, MR imaging can be used to obtain anatomic, functional, and metabolic information via volumetric and multiplanar imaging. Recently, MR imaging has been used in the planning, monitoring, and posttreatment assessment phases of LTSL drug delivery in combination with HIFU. Fig. 5 depicts the use of MR-HIFU in combination with LTSLs.
Treatment Planning
Treatment planning for hyperthermia-mediated drug delivery can be performed using MR images displayed within a graphical user interface (GUI) that provides tools for precise delineation of the target tissue ( Fig. 6 ). The treatment plan is directly overlaid on the anatomic images, defining, for example, lower and upper temperature thresholds at targeted locations. The GUI provides comprehensive visualization of the therapy plan and real-time progress, allowing for intraprocedural adjustments in response to changing conditions or new information acquired during the procedure.
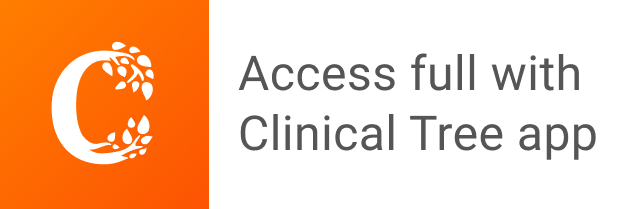