Magnetic resonance (MR) neurography – guided nerve blocks and injections describe a techniques for selective percutaneous drug delivery, in which limited MR neurography and interventional MR imaging are used jointly to map and target specific pelvic nerves or muscles, navigate needles to the target, visualize the injected drug and detect spread to confounding structures. The procedures described, specifically include nerve blocks of the obturator nerve, lateral femoral cutaneous nerve, pudendal nerve, posterior femoral cutaneous nerve, sciatic nerve, ganglion impar, sacral spinal nerve, and injection into the piriformis muscle.
Key points
- •
Magnetic resonance neurography (MRN)–guided interventional magnetic resonance imaging (MRI) describes a technique for selective nerve blocks, in which limited MRN and interventional MRI are used in combination.
- •
MRN-guided nerve pelvic blocks favorably combine the benefits of direct visualization of the nerve targets, objective assessment of the distribution of the injectant, and absence of ionizing radiation.
- •
MRN-guided nerve pelvic blocks achieve high face validity by directly visualizing the target, needle tip, injectant surrounding the nerve, and detection of spread to confounding structures.
- •
Selective MRN-guided nerve blocks are especially suited for deeply situated and small nerves, which are difficult to visualize and target with other imaging modalities.
- •
Selective MRN-guided nerve blocks can help diagnose and treat pelvic neuropathies causing chronic pelvic pain syndrome.
Introduction
Chronic pelvic pain is a multifactorial condition with a high prevalence in the general population and a substantial socioeconomic impact. The estimated prevalence of chronic pelvic pain in women is 3.8%, which is very similar to the prevalence of back pain. An estimated 15% to 20% of women between 18 and 50 years of age experience chronic pelvic pain, which accounts for up to 40% of patients visiting gynecologic outpatient clinics and $881.5 million dollars spent each year on outpatient management in the United States alone. The diagnostic workup and management frequently represent a major challenge to health care providers because of multiple possible causes and often poor response to treatment. Patients with chronic pelvic pain use 3 times more medication, have 4 times more nongynecologic surgeries, and are 5 times more likely to have hysterectomy. Chronic pelvic pain syndrome represents a subdivision of chronic pelvic pain, with no evidence of an infectious origin and no obvious causative local abnormality. The various causes of chronic pelvic pain syndrome include urologic, gynecologic, gastrointestinal, neurologic, and sexologic causes, and pelvic floor dysfunction. Chronicity may be defined as cyclic and noncyclic pain in women and men for at least 6 months localizing to the anatomic pelvis, anterior abdominal wall at or below the umbilicus, the lumbosacral back, or the buttocks, with associated functional disability, medical care, and negative cognitive, behavioral, sexual, and emotional consequences.
Peripheral nerve pain syndromes and pelvic neuropathies constitute an important group of disorders that can cause chronic pelvic pain syndrome. Causes include nerve compression, entrapment, repetitive microtrauma, impact injury, injection injury, surgical incisions, and postoperative scarring. Suggestive symptoms are paresthesia, dysesthesias, allodynia, dysreflexia, dyspareunia, and muscular dysfunction of the anatomic area innervated by the injured nerve. In addition to history, physical examination, and imaging, selective perineural injection with a local anesthetic (nerve block) is a helpful tool in the diagnostic workup of patients in whom a neuropathic origin of pain is suspected ( Table 1 ). Diagnostic nerve blocks are ordered to specifically test the hypothesis that a particular nerve is a source of pain. In cases of unsatisfactory response to conservative therapy, the results of nerve blocks may be used as a tool to help to guide future management. Additionally, locally delivered anti-inflammatory and anesthetic drugs may result in therapeutic benefit by interrupting pathways involved in nociceptor stimulation.
Diagnostic Nerve Block | Therapeutic Nerve Block |
---|---|
Clinically suspected neuropathy | Adjunct to conservative treatment |
Discordance of clinical findings and imaging (false-negative or false-positive results) | Inoperable condition |
Presurgical testing (eg, neurolysis and tumor resection in multiple lesions) | Expedite prolonged recovery after surgery |
Suspected failed surgery or recurrence | Induce atrophy of the muscle causing nerve compression |
Allergy to iodinated contrast agents | Failed nerve surgery or recurrence |
Contraindication or adverse effects to systemic pain medications/steroids and allergy to iodinated contrast agents | |
Small piriformis muscle (in a large patient) not adequately visualized or targeted with other techniques |
MR neurography (MRN)–guided interventional magnetic resonance imaging (MRI) is the combination of limited intraprocedural MRN and interventional MRI. MRN has the distinct ability to directly visualize and map the course of deep pelvic nerve targets. MRN-guided blocks are typically preceded by high-field MRN, which contributes helpful anatomic information for targeting. With the use of proper technique, the targeted anatomic structure, regional confounding structures, and the location and spread of the injected drug (injectant) can be directly visualized. MRN-guided nerve blocks, therefore, offer exquisite technical accuracy, allowing the interventionalist an objective assessment of the technical validity of the blocks. MRN guidance is especially suited for deeply situated neural targets (pudendal canal, obturator foramen) and small targets (ganglion impar, posterior femoral cutaneous nerve, intrapelvic lateral femoral cutaneous nerve proximal to the inguinal ligament, and neurogenic neoplasms), and where toxic injectants, such as botulinum neurotoxin, are being used. Additionally, MRN guidance is helpful in guiding presurgical planning because of the requirement of a high level of validity.
Ultrasound guidance is frequently adequate for superficial targets; however, deeply situated pelvic nerves are frequently not reliably visualized because of the lack of an acoustic window, limited contrast and spatial resolution, or insufficient penetration depth. Fluoroscopy can guide needle placements using bony landmarks; however, nerves cannot be visualized. Anatomic variants are frequently not seen, which may lead to nerve injury, and confounding spread to adjacent nerves may go unrecognized with fluoroscopy. Computed tomography (CT) guidance has similar limitations and also results in exposure to ionizing radiation. With the exception of the sciatic nerve and piriformis muscle, the low contrast resolution prevents accurate identification of the smaller peripheral nerves without the need for intravenous contrast administration.
MRI guidance obviates procedure-related ionizing radiation exposure of patients and operators, and its related health risks. The exact morbidity and mortality related to procedure-related ionizing radiation is not known; however, it was estimated that 1.5% to 2.0% of all U.S. population cancers may be caused by CT radiation exposure. Although the radiation dose of CT can be reduced using intermittent technique and low energy settings, MRN guidance is a preferable technique because of the absence of ionizing radiation. Interventional MRI complies with the ALARA (As Low As Reasonably Achievable) practice mandate in an exemplary fashion and is especially valuable in adolescents, young women of child-bearing age, and pregnant women. Cumulative radiation doses can be avoided, especially when serial nerve blocks are required. Additional attributes of MRN guidance are the unmatched soft tissue resolution for the differentiation and direct visualization of the target nerve, real-time needle MRI guidance at 1.5 T, easy visualization of the oblique needle paths because of multiplanar imaging capabilities, and real-time monitoring of injections. Because of factors such as cost and time requirements, however, MRN guidance is no substitute for ultrasound and CT guidance, but is rather a tool for selected cases, such as the targeting of and prevention of injury to small and deeply situated nerves for which only MRN guidance can provide the required technical accuracy from direct visualization.
This article discusses the technical background of MRN-guided interventional MRI, reviews important principles of nerve blocks, describes techniques of pelvic injection procedures, and illustrates targets and procedures for the diagnosis and therapy of neurogenic chronic pelvic pain syndrome, in which MRN guidance provides exquisite detail for valid nerve blocks.
MR Imaging Systems
Although MRN-guided interventions may be performed with any MRI system, the introduction of clinical 1.5-T MRI systems with a wide-bore magnet design has greatly improved the utility of MRN-guided injections through obviating the requirement of a dedicated, low-field open magnetic resonance (MR) imaging systems, which were previously used. The wide bore of the magnet allows improved patient access and needle maneuvering while the patient is in the isocenter of the bore of the magnet, and simultaneous image acquisition and review. Patient access and the spatial properties are similar to those of current dual-source CT imaging systems.
The use of 1.5-T field-strength magnets, modern coil design and parallel imaging technology can result in less signal averaging and faster image acquisition and display and has advanced the field of MRI-guided and MRN-guided interventions. Because the MR signal increases with the strength of the magnet, MRN-guided injections at 1.5 T provide substantially higher signal-to-noise ratios when compared with low-field MRI systems of 0.2- to 0.5-T strength. The higher MR signal permits higher spatial resolution and 3-dimensional isotropic MR imaging, which allow direct visualization of small nerves and peripheral branches of the lumbosacral plexus, which is a prerequisite for direct targeting. Additionally, higher bandwidths can be used, which are beneficial for accurate needle tip visualization through passive needle artifact creation. Because of proportionally increasing chemical shift, the reliable use of spectral fat saturation techniques is possible, which increases the visibility and detectability of the injected drugs. A field strength of 1.5 T also allows the use of real-time fluoroscopic MR imaging with high spatial and temporal resolution. MR fluoroscopy describes the continuous acquisition and display of MR images, similar to CT fluoroscopy, which can be used for interactive device handling.
Safety
The 1.5-T external magnetic field, radiofrequency pulses, and gradients contribute to the environment of an interventional MR suite, similar to diagnostic MRI. Therefore, effective screening procedures are required to guard the safety of MR interventions and avoid accidents. In addition to the general contraindications of MRI, pacemakers and pregnancy require careful consideration. The ferromagnetic devices or equipment traditionally used in interventional radiology must not be brought into the MR environment because they are prone to experience considerable traction forces that may be strong enough to cause serious fatalities, or significant heating that might result in burn injuries to patients and physicians. Therefore, conventional stainless steel injection needles cannot be used safely for MRN-guided injections. Today, a variety of MR-compatible needles are commercially available from several vendors for use at 1.5 T.
Needle Visualization
The constant and reliable visualization of the injection needle is a prerequisite for effective and safe percutaneous MR-guided drug delivery. In contrast to conventional radiographic fluoroscopy or CT, which both use attenuation differences of x-rays to visualize the needle, the mechanisms of accurate needle visualization are more complex in interventional MR imaging. In addition to the certified MR compatibility of the needle, spatial misregistration and image distortions must be avoided. The size of the created needle artifact should be large enough to be detectable on MR images but should avoid overlapping with adjacent structures, and the overestimation of the length of the needle should be minimal. With optimized pulse sequences, the passive needle artifact displays the true location of the needle tip with an error margin of only 1 mm at 1.5 T. Of the various techniques for localization and display of the needle tip on MR images, passive visualization has proven to be an easy and effective method because no additional equipment, recalculation, or postprocessing of MR images is needed. The needle artifact is predominantly created by localized spin dephasing, which results from regional field inhomogeneities caused by low magnetic susceptibility alloys of MR-compatible needles and by local gradient disturbances.
Aside from the composition of the needle alloy, needle geometry, and field strength, several variable factors and parameters relevant to the appearance of the needle artifact exist. Familiarity with the practical implications of factors, such as type of pulse sequence, specific parameters (echo time, voxel size, readout and radiofrequency bandwidth), and the relative angle of the needle to the orientation of the external magnetic field influence the needle artifact and enable the operator to optimize the appearance of the needle artifact appearance. In contrast to spin echo and turbo spin echo sequences, gradient echo sequences amplify the needle artifact because of the build-up of field heterogeneities. This effect is more pronounced at 1.5 T. When using gradient echo sequences, minimization of the echo times results in shortening of the time for dephasing and a smaller needle artifact. Although dephasing is less pronounced with turbo spin echo sequences, a similar benefit may result from shortening of echo times and the use of short echo spacing. Maximizing bandwidths results in similar effects. Maximization of gradient strengths minimizes susceptibility artifacts, which can also be achieved using a small field of view and small slice thickness. The needle artifact is strongest if the needle is perpendicularly oriented to the external magnetic field, whereas it is smallest if oriented parallel. When using an MRI system with a horizontal bore, most injections are performed in the axial plane and therefore the needle is generally oriented 90° to the external magnetic field and parallel to the patient’s z-axis.
Visualization of Injectants
Reliable visualization of the deposition and spread of injectants is fundamental to judge the technical adequacy of a nerve block and its validity in terms of patient’s pain response. Injectants can be visualized with T1-weighted or T2-weighted MR imaging. The addition of Gadolinium-based contrast to the injectant enables its selective visualization with the use of T1-weighted sequences and fat saturation. The addition of gadolinium-based contrast agent (eg, gadolinium-DTPA) in a ratio of 1:300 results in high contrast-to-noise ratios between the injectant and the surrounding tissues. Injectants may also be visualized with short tau inversion recovery (STIR) imaging or T2-weighted sequences with optional fat saturation, based on the intrinsically long T2 of the water-based injectant. In our experience, both techniques can be equally effective. Gadolinium-enhanced injectants are useful for real-time MR fluoroscopic monitoring of the injection and detection of perineural spread when using T1-weighted MR fluoroscopy sequences ( Fig. 1 ). The safety of perineural injection of gadolinium-based contrast material with the combination of anesthetic and steroids has been confirmed by several investigations. T2-weighted visualization may be preferred because it obviates the addition of gadolinium-based contrast. Either approach can be advantageous in treating patients with hypersensitivity to iodine, for whom iodine-based contrast agents, such as those used in CT and radiographic fluoroscopy are contraindicated.
MR Fluoroscopy
MR fluoroscopy is useful for interactive determination of the skin entry site, needle advancement navigation, and real-time monitoring of injections, which can be viewed on an MR-compatible in-room display (see Fig. 1 ). The required high temporal resolution results in a trade-off between spatial and contrast resolution. However, both spoiled and balanced gradient echo sequences achieve frame rates of less than 1 second with sufficient spatial and contrast resolution.
Protocol
Parallel imaging with the use of surface coils and the table coil elements achieves rapid image acquisition and can increase the signal-to-noise ratio of the target region, as opposed to the use of the body coil, which is installed in the bore of the magnet. In each procedure, limited MRN images are acquired first to map the target nerve. A multichannel surface coil placed over the interventional site is advantageous to achieve the highest resolution. For the needle placement, the multichannel surface coil may be exchanged for a surface loop coil, which facilitates free access to the interventional site and sterile coverage.
All procedures discussed in this article were performed with a clinical, open-bore 1.5-T MRI system (Magnetom Espree, Siemens Healthcare, Erlangen, Germany) following a 6-step algorithm ( Table 2 ). Depending on the target, patients are placed either prone or supine on the MR table, depending on the location the target ( Table 3 ). T1-weighted MRN images are obtained immediately before the needle placement phase using a multichannel body coil placed over the pelvis ( Table 4 , sequence 1). On these images, the operator maps the target structure from the level of its origin to the periphery, and the needle path is planned on the work station depending on the indication for injection. The coil is then exchanged with a flexible loop-shaped radiofrequency coil with a diameter of 16 cm (Siemens Healthcare). The skin entry point is determined interactively using MR fluoroscopy (see Table 4 , sequence 2) using a water-filled syringe as a pointing device or the operator’s fingertip with the MR fluoroscopy sequence prescribed to the slice position of the selected injection site. The interventional site is then prepped and draped in standard fashion. Conscious sedation may be initiated at this point according to the patient’s preference and the procedure type. However, because the described procedures are simple, with an average procedure time of 30 minutes, the authors prefer not to give sedation in order to not confound the pain response. Local anesthesia is administered superficially using 1% lidocaine through a 25-G hypodermic needle. MR-compatible 22- or 20-G injection needles of 10- or 15-cm lengths (MReye, G11583, Cook Medical, Bloomington, IN, USA), depending on the skin-to-target distance, are then interactively navigated to the target under MR fluoroscopy guidance. Intermittent axial turbo spin echo MR images (see Table 4 , sequence 3) are acquired for visual assessment of the adequacy of the needle tip location. The administration of gadolinium-enhanced injectant is monitored using T1-weighted MR fluoroscopy, whereas nonenhanced injections are monitored with a fast STIR sequence (see Table 4 , sequence 4). The location of the delivered injectant is finally visualized using a fat-saturated T1-weighted turbo spin echo MR sequence (see Table 4 , sequence 5) or an isotropic 3-dimensional T2-weighted MR imaging sequence with or without fat saturation (see Table 4 , sequence 6).
Step | Action |
---|---|
1 | Limited MRN visualization of the target muscle or nerve, mapping of its course, and planning of the needle path. |
2 | MR fluoroscopy for the determination of a suitable skin entry point using the tip of a syringe as the pointing device or the operator’s finger. |
3 | Needle placement to the target navigated by MR fluoroscopy or intermittent MR imaging. |
4 | Confirmation of the adequacy of the location of the needle tip using fast intermediate-weighted MR imaging. |
5 | Monitoring of the injection by MR fluoroscopy or intermittent MR imaging with optional preceding sterile saline test injection. |
6 | Final MR imaging for visualizing the injectant, determining the spatial relationship to the target structure, and detecting potential spread to adjacent structures. |
Anterior | Posterior |
---|---|
Lateral femoral cutaneous nerve | Posterior femoral cutaneous nerve |
Femoral nerve | Sciatic nerve |
Obturator nerve | Pudendal nerve |
Ganglion impar | |
Piriformis injection | |
Hypogastric plexus | |
Sacral foraminal injection |
Sequence | ||||||
---|---|---|---|---|---|---|
1 | 2 | 3 | 4 | 5 | 6 | |
Type | Turbo spin echo | FLASH2D | Turbo spin echo | STIR | Turbo spin echo | SPACE |
Weighting | T1 | T1/T2* | Intermediate | STIR | T1 | T2 |
Fat-saturation | No | No | No | Yes | Yes | Optional |
Dimensionality | 2D | 2D | 2D | 2D | 2D | 3D |
Orientation | Axial | Axial | Axial | Axial | Axial | Coronal |
Repetition time (ms) | 691.0 | 9.3 | 1200.0 | 1200.0 | 500.0 | 1500.0 |
Echo time (ms) | 20.0 | 3.5 | 12.0 | 29.0 | 20.0 | 138.0 |
Echo train length | 5 | NA | 17 | 5 | 6 | 8 |
Slice thickness (mm) | 4 | 5 | 5 | 5 | 4 | 1 |
Field of view (mm) | 360 × 253 | 256 × 224 | 256 × 224 | 256 × 224 | 200 × 200 | 300 × 207 |
Base resolution (pixels) | 384 | 256 | 320 | 192 | 384 | 384 |
Phase resolution (%) | 70 | 56 | 100 | 45 | 50 | 74 |
Receiver bandwidth (Hz) | 102 | 180 | 252 | 542 | 102 | 751 |
Acquisition time | 3.6 min | 1 s/frame | 12 s | 32 s | 5.1 min | 6–7 min |
Introduction
Chronic pelvic pain is a multifactorial condition with a high prevalence in the general population and a substantial socioeconomic impact. The estimated prevalence of chronic pelvic pain in women is 3.8%, which is very similar to the prevalence of back pain. An estimated 15% to 20% of women between 18 and 50 years of age experience chronic pelvic pain, which accounts for up to 40% of patients visiting gynecologic outpatient clinics and $881.5 million dollars spent each year on outpatient management in the United States alone. The diagnostic workup and management frequently represent a major challenge to health care providers because of multiple possible causes and often poor response to treatment. Patients with chronic pelvic pain use 3 times more medication, have 4 times more nongynecologic surgeries, and are 5 times more likely to have hysterectomy. Chronic pelvic pain syndrome represents a subdivision of chronic pelvic pain, with no evidence of an infectious origin and no obvious causative local abnormality. The various causes of chronic pelvic pain syndrome include urologic, gynecologic, gastrointestinal, neurologic, and sexologic causes, and pelvic floor dysfunction. Chronicity may be defined as cyclic and noncyclic pain in women and men for at least 6 months localizing to the anatomic pelvis, anterior abdominal wall at or below the umbilicus, the lumbosacral back, or the buttocks, with associated functional disability, medical care, and negative cognitive, behavioral, sexual, and emotional consequences.
Peripheral nerve pain syndromes and pelvic neuropathies constitute an important group of disorders that can cause chronic pelvic pain syndrome. Causes include nerve compression, entrapment, repetitive microtrauma, impact injury, injection injury, surgical incisions, and postoperative scarring. Suggestive symptoms are paresthesia, dysesthesias, allodynia, dysreflexia, dyspareunia, and muscular dysfunction of the anatomic area innervated by the injured nerve. In addition to history, physical examination, and imaging, selective perineural injection with a local anesthetic (nerve block) is a helpful tool in the diagnostic workup of patients in whom a neuropathic origin of pain is suspected ( Table 1 ). Diagnostic nerve blocks are ordered to specifically test the hypothesis that a particular nerve is a source of pain. In cases of unsatisfactory response to conservative therapy, the results of nerve blocks may be used as a tool to help to guide future management. Additionally, locally delivered anti-inflammatory and anesthetic drugs may result in therapeutic benefit by interrupting pathways involved in nociceptor stimulation.
Diagnostic Nerve Block | Therapeutic Nerve Block |
---|---|
Clinically suspected neuropathy | Adjunct to conservative treatment |
Discordance of clinical findings and imaging (false-negative or false-positive results) | Inoperable condition |
Presurgical testing (eg, neurolysis and tumor resection in multiple lesions) | Expedite prolonged recovery after surgery |
Suspected failed surgery or recurrence | Induce atrophy of the muscle causing nerve compression |
Allergy to iodinated contrast agents | Failed nerve surgery or recurrence |
Contraindication or adverse effects to systemic pain medications/steroids and allergy to iodinated contrast agents | |
Small piriformis muscle (in a large patient) not adequately visualized or targeted with other techniques |
MR neurography (MRN)–guided interventional magnetic resonance imaging (MRI) is the combination of limited intraprocedural MRN and interventional MRI. MRN has the distinct ability to directly visualize and map the course of deep pelvic nerve targets. MRN-guided blocks are typically preceded by high-field MRN, which contributes helpful anatomic information for targeting. With the use of proper technique, the targeted anatomic structure, regional confounding structures, and the location and spread of the injected drug (injectant) can be directly visualized. MRN-guided nerve blocks, therefore, offer exquisite technical accuracy, allowing the interventionalist an objective assessment of the technical validity of the blocks. MRN guidance is especially suited for deeply situated neural targets (pudendal canal, obturator foramen) and small targets (ganglion impar, posterior femoral cutaneous nerve, intrapelvic lateral femoral cutaneous nerve proximal to the inguinal ligament, and neurogenic neoplasms), and where toxic injectants, such as botulinum neurotoxin, are being used. Additionally, MRN guidance is helpful in guiding presurgical planning because of the requirement of a high level of validity.
Ultrasound guidance is frequently adequate for superficial targets; however, deeply situated pelvic nerves are frequently not reliably visualized because of the lack of an acoustic window, limited contrast and spatial resolution, or insufficient penetration depth. Fluoroscopy can guide needle placements using bony landmarks; however, nerves cannot be visualized. Anatomic variants are frequently not seen, which may lead to nerve injury, and confounding spread to adjacent nerves may go unrecognized with fluoroscopy. Computed tomography (CT) guidance has similar limitations and also results in exposure to ionizing radiation. With the exception of the sciatic nerve and piriformis muscle, the low contrast resolution prevents accurate identification of the smaller peripheral nerves without the need for intravenous contrast administration.
MRI guidance obviates procedure-related ionizing radiation exposure of patients and operators, and its related health risks. The exact morbidity and mortality related to procedure-related ionizing radiation is not known; however, it was estimated that 1.5% to 2.0% of all U.S. population cancers may be caused by CT radiation exposure. Although the radiation dose of CT can be reduced using intermittent technique and low energy settings, MRN guidance is a preferable technique because of the absence of ionizing radiation. Interventional MRI complies with the ALARA (As Low As Reasonably Achievable) practice mandate in an exemplary fashion and is especially valuable in adolescents, young women of child-bearing age, and pregnant women. Cumulative radiation doses can be avoided, especially when serial nerve blocks are required. Additional attributes of MRN guidance are the unmatched soft tissue resolution for the differentiation and direct visualization of the target nerve, real-time needle MRI guidance at 1.5 T, easy visualization of the oblique needle paths because of multiplanar imaging capabilities, and real-time monitoring of injections. Because of factors such as cost and time requirements, however, MRN guidance is no substitute for ultrasound and CT guidance, but is rather a tool for selected cases, such as the targeting of and prevention of injury to small and deeply situated nerves for which only MRN guidance can provide the required technical accuracy from direct visualization.
This article discusses the technical background of MRN-guided interventional MRI, reviews important principles of nerve blocks, describes techniques of pelvic injection procedures, and illustrates targets and procedures for the diagnosis and therapy of neurogenic chronic pelvic pain syndrome, in which MRN guidance provides exquisite detail for valid nerve blocks.
MR Imaging Systems
Although MRN-guided interventions may be performed with any MRI system, the introduction of clinical 1.5-T MRI systems with a wide-bore magnet design has greatly improved the utility of MRN-guided injections through obviating the requirement of a dedicated, low-field open magnetic resonance (MR) imaging systems, which were previously used. The wide bore of the magnet allows improved patient access and needle maneuvering while the patient is in the isocenter of the bore of the magnet, and simultaneous image acquisition and review. Patient access and the spatial properties are similar to those of current dual-source CT imaging systems.
The use of 1.5-T field-strength magnets, modern coil design and parallel imaging technology can result in less signal averaging and faster image acquisition and display and has advanced the field of MRI-guided and MRN-guided interventions. Because the MR signal increases with the strength of the magnet, MRN-guided injections at 1.5 T provide substantially higher signal-to-noise ratios when compared with low-field MRI systems of 0.2- to 0.5-T strength. The higher MR signal permits higher spatial resolution and 3-dimensional isotropic MR imaging, which allow direct visualization of small nerves and peripheral branches of the lumbosacral plexus, which is a prerequisite for direct targeting. Additionally, higher bandwidths can be used, which are beneficial for accurate needle tip visualization through passive needle artifact creation. Because of proportionally increasing chemical shift, the reliable use of spectral fat saturation techniques is possible, which increases the visibility and detectability of the injected drugs. A field strength of 1.5 T also allows the use of real-time fluoroscopic MR imaging with high spatial and temporal resolution. MR fluoroscopy describes the continuous acquisition and display of MR images, similar to CT fluoroscopy, which can be used for interactive device handling.
Safety
The 1.5-T external magnetic field, radiofrequency pulses, and gradients contribute to the environment of an interventional MR suite, similar to diagnostic MRI. Therefore, effective screening procedures are required to guard the safety of MR interventions and avoid accidents. In addition to the general contraindications of MRI, pacemakers and pregnancy require careful consideration. The ferromagnetic devices or equipment traditionally used in interventional radiology must not be brought into the MR environment because they are prone to experience considerable traction forces that may be strong enough to cause serious fatalities, or significant heating that might result in burn injuries to patients and physicians. Therefore, conventional stainless steel injection needles cannot be used safely for MRN-guided injections. Today, a variety of MR-compatible needles are commercially available from several vendors for use at 1.5 T.
Needle Visualization
The constant and reliable visualization of the injection needle is a prerequisite for effective and safe percutaneous MR-guided drug delivery. In contrast to conventional radiographic fluoroscopy or CT, which both use attenuation differences of x-rays to visualize the needle, the mechanisms of accurate needle visualization are more complex in interventional MR imaging. In addition to the certified MR compatibility of the needle, spatial misregistration and image distortions must be avoided. The size of the created needle artifact should be large enough to be detectable on MR images but should avoid overlapping with adjacent structures, and the overestimation of the length of the needle should be minimal. With optimized pulse sequences, the passive needle artifact displays the true location of the needle tip with an error margin of only 1 mm at 1.5 T. Of the various techniques for localization and display of the needle tip on MR images, passive visualization has proven to be an easy and effective method because no additional equipment, recalculation, or postprocessing of MR images is needed. The needle artifact is predominantly created by localized spin dephasing, which results from regional field inhomogeneities caused by low magnetic susceptibility alloys of MR-compatible needles and by local gradient disturbances.
Aside from the composition of the needle alloy, needle geometry, and field strength, several variable factors and parameters relevant to the appearance of the needle artifact exist. Familiarity with the practical implications of factors, such as type of pulse sequence, specific parameters (echo time, voxel size, readout and radiofrequency bandwidth), and the relative angle of the needle to the orientation of the external magnetic field influence the needle artifact and enable the operator to optimize the appearance of the needle artifact appearance. In contrast to spin echo and turbo spin echo sequences, gradient echo sequences amplify the needle artifact because of the build-up of field heterogeneities. This effect is more pronounced at 1.5 T. When using gradient echo sequences, minimization of the echo times results in shortening of the time for dephasing and a smaller needle artifact. Although dephasing is less pronounced with turbo spin echo sequences, a similar benefit may result from shortening of echo times and the use of short echo spacing. Maximizing bandwidths results in similar effects. Maximization of gradient strengths minimizes susceptibility artifacts, which can also be achieved using a small field of view and small slice thickness. The needle artifact is strongest if the needle is perpendicularly oriented to the external magnetic field, whereas it is smallest if oriented parallel. When using an MRI system with a horizontal bore, most injections are performed in the axial plane and therefore the needle is generally oriented 90° to the external magnetic field and parallel to the patient’s z-axis.
Visualization of Injectants
Reliable visualization of the deposition and spread of injectants is fundamental to judge the technical adequacy of a nerve block and its validity in terms of patient’s pain response. Injectants can be visualized with T1-weighted or T2-weighted MR imaging. The addition of Gadolinium-based contrast to the injectant enables its selective visualization with the use of T1-weighted sequences and fat saturation. The addition of gadolinium-based contrast agent (eg, gadolinium-DTPA) in a ratio of 1:300 results in high contrast-to-noise ratios between the injectant and the surrounding tissues. Injectants may also be visualized with short tau inversion recovery (STIR) imaging or T2-weighted sequences with optional fat saturation, based on the intrinsically long T2 of the water-based injectant. In our experience, both techniques can be equally effective. Gadolinium-enhanced injectants are useful for real-time MR fluoroscopic monitoring of the injection and detection of perineural spread when using T1-weighted MR fluoroscopy sequences ( Fig. 1 ). The safety of perineural injection of gadolinium-based contrast material with the combination of anesthetic and steroids has been confirmed by several investigations. T2-weighted visualization may be preferred because it obviates the addition of gadolinium-based contrast. Either approach can be advantageous in treating patients with hypersensitivity to iodine, for whom iodine-based contrast agents, such as those used in CT and radiographic fluoroscopy are contraindicated.
MR Fluoroscopy
MR fluoroscopy is useful for interactive determination of the skin entry site, needle advancement navigation, and real-time monitoring of injections, which can be viewed on an MR-compatible in-room display (see Fig. 1 ). The required high temporal resolution results in a trade-off between spatial and contrast resolution. However, both spoiled and balanced gradient echo sequences achieve frame rates of less than 1 second with sufficient spatial and contrast resolution.
Protocol
Parallel imaging with the use of surface coils and the table coil elements achieves rapid image acquisition and can increase the signal-to-noise ratio of the target region, as opposed to the use of the body coil, which is installed in the bore of the magnet. In each procedure, limited MRN images are acquired first to map the target nerve. A multichannel surface coil placed over the interventional site is advantageous to achieve the highest resolution. For the needle placement, the multichannel surface coil may be exchanged for a surface loop coil, which facilitates free access to the interventional site and sterile coverage.
All procedures discussed in this article were performed with a clinical, open-bore 1.5-T MRI system (Magnetom Espree, Siemens Healthcare, Erlangen, Germany) following a 6-step algorithm ( Table 2 ). Depending on the target, patients are placed either prone or supine on the MR table, depending on the location the target ( Table 3 ). T1-weighted MRN images are obtained immediately before the needle placement phase using a multichannel body coil placed over the pelvis ( Table 4 , sequence 1). On these images, the operator maps the target structure from the level of its origin to the periphery, and the needle path is planned on the work station depending on the indication for injection. The coil is then exchanged with a flexible loop-shaped radiofrequency coil with a diameter of 16 cm (Siemens Healthcare). The skin entry point is determined interactively using MR fluoroscopy (see Table 4 , sequence 2) using a water-filled syringe as a pointing device or the operator’s fingertip with the MR fluoroscopy sequence prescribed to the slice position of the selected injection site. The interventional site is then prepped and draped in standard fashion. Conscious sedation may be initiated at this point according to the patient’s preference and the procedure type. However, because the described procedures are simple, with an average procedure time of 30 minutes, the authors prefer not to give sedation in order to not confound the pain response. Local anesthesia is administered superficially using 1% lidocaine through a 25-G hypodermic needle. MR-compatible 22- or 20-G injection needles of 10- or 15-cm lengths (MReye, G11583, Cook Medical, Bloomington, IN, USA), depending on the skin-to-target distance, are then interactively navigated to the target under MR fluoroscopy guidance. Intermittent axial turbo spin echo MR images (see Table 4 , sequence 3) are acquired for visual assessment of the adequacy of the needle tip location. The administration of gadolinium-enhanced injectant is monitored using T1-weighted MR fluoroscopy, whereas nonenhanced injections are monitored with a fast STIR sequence (see Table 4 , sequence 4). The location of the delivered injectant is finally visualized using a fat-saturated T1-weighted turbo spin echo MR sequence (see Table 4 , sequence 5) or an isotropic 3-dimensional T2-weighted MR imaging sequence with or without fat saturation (see Table 4 , sequence 6).
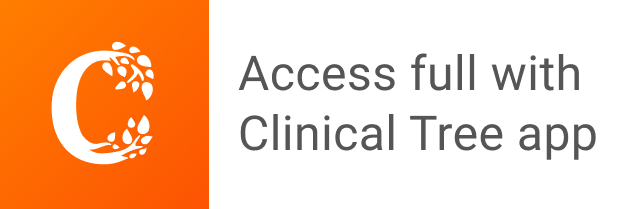