Fig. 3.1
Macrophage imaging in hyperlipidemic rabbits 6 months after balloon injury of the aorta. (a) MR images taken 72 h after intravenous administration of the USPIO MION-47. MION-47 administration decreased T2 signals in the aorta (upper right), but did not affect T1 signals (lower right). (b) Effects of lipid-lowering therapy by rosuvastatin on T2 signal reductions. MION-47-induced reduction of T2 signals was decreased by 3 months of rosuvastatin treatment. (c) Correlation between T2 signal reduction in MRI and histologically determined macrophage accumulation (Edited from Ref. [7])
These iron-based nanoparticles could be modified with various proteins or fluorescences. USPIO modified with peptidic scavenger receptor-AI (SR-AI) ligand showed 3.5-fold higher accumulation into atherosclerotic plaques compared to nonconjugated USPIO [8]. Lectin-like oxLDL receptor 1 (LOX-1)-targeted USPIO co-localizes with LOX-1, macrophages, and MMP-9 in atherosclerotic plaque of apolipoprotein E-deficient (ApoE−/−) mice [9]. USPIO already has been tested in humans and reported to be useful to monitor the effects of statins in carotid artery plaques [10, 11].
How these small nanoparticles are taken up by tissue macrophages or peripheral blood monocytes remains incompletely understood. Relatively large particles are taken up by phagocytosis, but the uptake of small-sized (<100 nm) particles may involve other mechanisms. For example, the uptake of cross-linked dextran iron oxide nanoparticles (CLIO) is not inhibited by phagocytosis inhibitor, cytochalasin D, but inhibited by macropinocytosis inhibitor, amiloride [5, 12]. These results suggest that these particles are taken up by macropinocytosis by monocytes/macrophages.
3.5 Macrophage Imaging by Nuclear Medicine
Nuclear medicine is one the promising modalities to visualize macrophage burden and activity in atherosclerotic plaques in patients and has dominated the field of clinical molecular imaging. PET with 18F-fluorodeoxyglucose (FDG-PET) visualizes cells with active glucose metabolism, although a recent study challenged this theory by demonstrating that hypoxia promotes macrophage uptake of FDG [13]. Validation studies used histological assessment to positively correlate high FDG signals with greater macrophage accumulation [14]. Clinical studies have established that FDG-PET monitors therapeutic effects of various drugs on macrophage-rich carotid arteries, suggesting that FDG serves as a biomarker of the inflammatory burden of atherosclerotic plaques [15–18]. Interestingly, one of these studies reported that FDG-PET detected a reduction of FDG uptake as early as 4 weeks after statin treatment. The advantage that 18FDG systemically circulates enables imaging of various tissues in the same patient. Bucerius et al. reported significant correlations between obesity, fat tissue inflammation, and vascular inflammation by imaging fat tissue and carotid/ascending arteries at the same time [19]. In several reports on diabetic patients, pioglitazone, an agonist of peroxisome proliferator-activated receptor-γ (PPAR-γ), reduced inflammation evaluated by FDG-PET/CT in human coronary arteries or aorta [20, 21]. Arterial FDG uptake is affected by blood glucose level before scanning and FDG circulation time [22]. Therefore, imaging protocols should be optimized for each target vessel to obtain sufficient 18FDG signals.
Preclinical research has continued to actively study FDG-PET. For example, Nishimura et al. compared images of intravascular ultrasound (IVUS) and FDG-PET with histological images and reported that FDG-PET can detect foamy macrophages in atherosclerotic plaques in Watanabe heritable hyperlipidemic (WHHL) rabbits [23]. Vucic et al. reported that FDG-PET/CT evaluated anti-inflammatory effects of liver X receptor (LXR) agonist R211945 in hyperlipidemic New Zealand white rabbits [24]. Millon et al. compared sensitivity of FDG-PET and MRI with USPIO. They monitored the effects of atorvastatin on vascular inflammation and found that FDG-PET is more sensitive to detect early changes in atherosclerotic lesions [25].
Active research also has focused on the development of new FDG agents. 11C-PK11195, which binds to peripheral benzodiazepine receptors (PBRs) expressed by macrophages, has been tested in a clinical trial using PET-CT as an imaging agent for plaque macrophages [26]. The study involves patients with symptomatic or asymptomatic carotid stenosis. Signals were correlated with macrophage content in carotid endarterectomy specimens and higher in symptomatic stenosis. Furthermore, new radionuclides targeting mannose receptor are reported [27]. Mannose receptors expressed on a subset of the macrophage population in high-risk plaques may be good targets for macrophage imaging. 2-deoxy-2-[18F]fluoro-D-mannose (18F-FDM) were taken up by cultured macrophages more efficiently than 18F-FDG, suggesting that 18F-FDM serves as a novel macrophage-targeting tracer [27]. Beer et al. reported PET/CT imaging which targets integrin αvβ3. [18F]Galacto-RGD, a novel tracer specifically binding to αvβ3 integrin, increased the target/background ratio in human carotid arteries with stenosis [28]. The target/background ratio significantly correlated with histologically examined αvβ3 expression. Seo et al. reported a novel tracer 64Cu-Labeled LyP-1-Dendrimer. LyP-1, a cyclic 9-amino acid peptide, recognizes and binds to p32 proteins on activated macrophages. This tracer accumulated in atherosclerotic plaques in ApoE−/−mice [29]. Hatori et al. reported N-benzyl-N-methyl-2-[7,8-dihydro-7-(2-[18F]fluoroethyl)-8-oxo-2-phenyl-9H-purin-9-yl]acetamide([18F]FEDAC). This tracer is delivered to macrophages and neutrophils in a rat acute liver damage model [30].
3.6 Imaging of Macrophage-Derived Proteases in Atherosclerotic Plaques
Extracellular matrix such as collagen enables atherosclerotic plaques to withstand hemodynamic stresses. Matrix metalloproteinase (MMP) and cathepsins, mainly produced by plaque macrophages or smooth muscle cells, may promote plaque instability by degrading extracellular matrix. Therefore, imaging of protease activity should help to screen high-risk vessels in clinical settings. Enzyme-activatable NIRF imaging probes (excitation/emission wavelength between 600 and 900 nm) have been used to visualize proteolytic activity. Such probes have a protease-specific quenched substrate, inserted between fluorescence and carrier vehicle. Once the substrate is cleaved by target proteinases (MMP, cathepsin, etc.), the probe elaborates NIRF signals (activated state). Due to its tissue penetration, NIRF imaging has been developed to visualize inflammation in deep tissues. We used mouse models of atherosclerosis to demonstrate the feasibility of visualizing macrophage-derived MMP activity and evaluating changes due to the pharmacological inhibition (Fig. 3.2) [2, 31]. The evidence suggests that macrophage accumulation promotes cardiovascular calcification, as established by molecular imaging [32]. NIRF imaging can co-map macrophages and osteoblastic activity and evaluate the effects of genetic manipulations or pharmacologic interventions (Fig. 3.3) [33, 34]. More recently, the Jaffer and Tearney groups have developed an intravascular molecular imaging modality [35, 36]. This technology involves a catheter-assisted dual imaging approach – optical frequency domain imaging (OFDI) and NIRF imaging. This dual-modality intra-arterial catheter can visualize atherosclerotic plaques with high protease activity in a hyperlipidemic rabbit model.
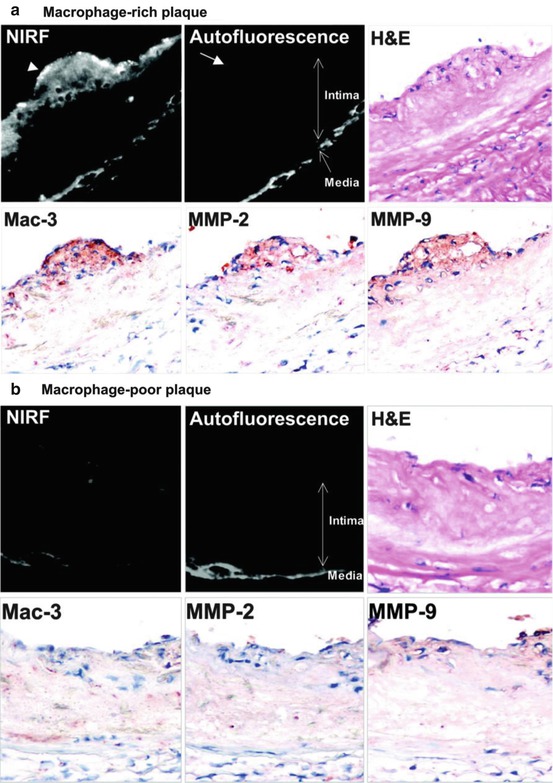
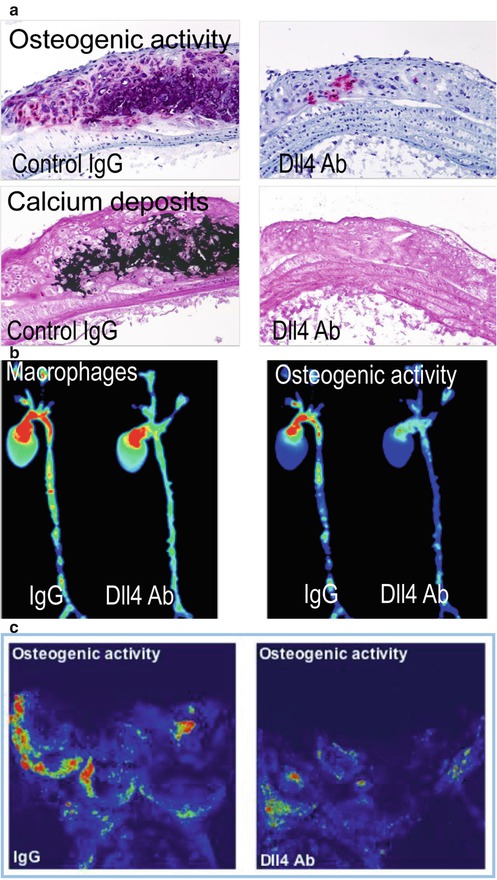
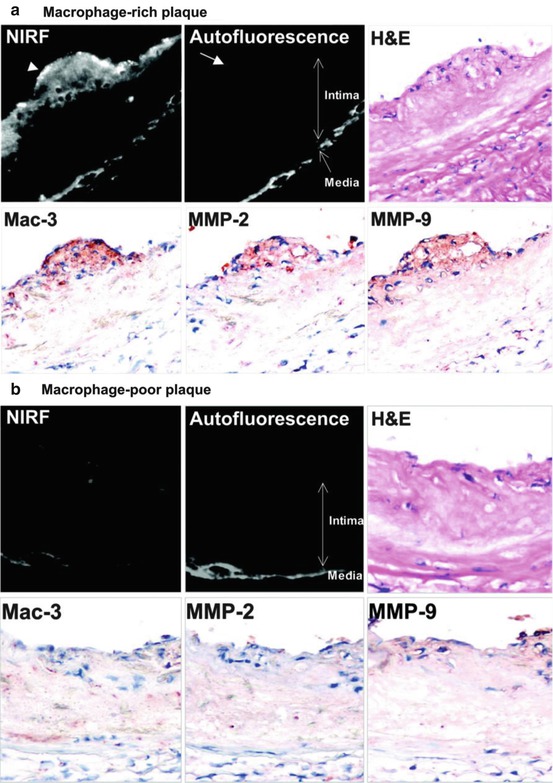
Fig. 3.2
Visualization of MMP activity by NIRF imaging in the aorta of ApoE−/− mice. Imaging agent, which is activated by gelatinase (MMP-2, MMP-9) and emits NIRF signal was injected 24 h before imaging. (a) In a macrophage-rich plaque, NIRF signal was detected in Mac-3-positive macrophages (arrowhead). Autofluorescence was observed in the media, but not in the intima (arrow). (b) NIRF imaging in a macrophage-poor plaque (Cited from Ref. [2])
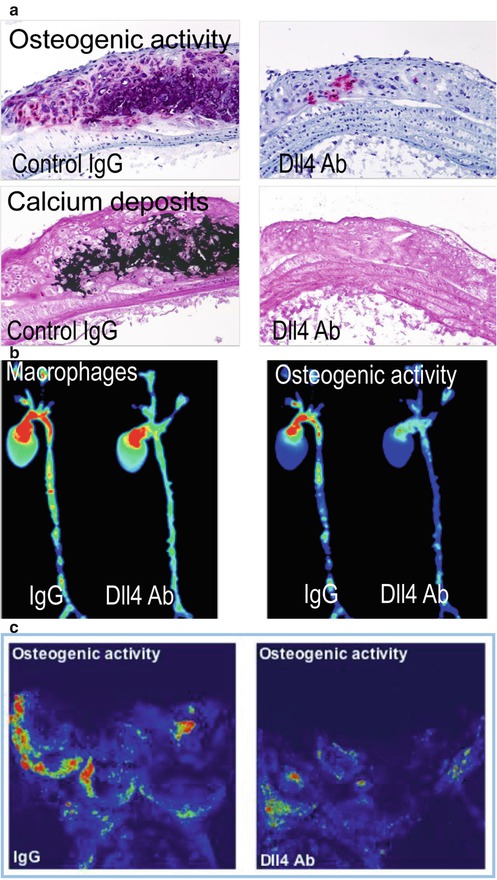
Fig. 3.3
Antibody blockade of the Notch ligand Delta-like 4 (Dll4) reduces aortic and valvular calcification in Ldlr−/− mice. (a) Histological assays for alkaline phosphatase activity (top) and calcium deposit demonstrate that Dll4 antibody administration reduced calcification in the aortas of fat-fed Ldl−/− mice. (b) NIRF imaging co-localized macrophages and osteogenic activity in excised aortas of Ldlr−/− mice. Dll4 blockade diminished macrophage accumulation and osteogenic activity in parallel. (c) Dll4 suppression reduced osteogenic activity in aortic valves of Ldl−/− mice (Cited from Ref. [34])
3.7 Imaging of Plaque Macrophages by HDL-Based Probes
High-density lipoprotein (HDL) mediates cholesterol efflux from cholesterol ester-enriched plaque macrophages and transfers the excessive cholesterol to the liver for excretion [37]. Therefore, HDL-based imaging agents have a possibility to visualize macrophages in atherosclerotic plaques. Macrophage-specific MRI contrast agent based on Gd-modified HDL was tested in ApoE−/− mice [38]. This agent accumulated in atherosclerotic plaque in the aorta 24 h after administration. Marrache et al. used a biodegradable HDL nanoparticle platform for the detection of macrophage apoptosis by targeting the collapse of mitochondrial membrane potential that occurs during apoptosis [39]. This particle contains quantum dots and is intended to apply for optical imaging.
3.8 Macrophage Imaging in Other Cardiovascular Diseases
Active research has attempted to establish imaging modalities for inflammation in various other cardiovascular diseases. The pathogenesis of aortic abdominal aneurysms (AAA) involves accumulation of activated macrophages. Macrophage imaging in patients may thus help identify high-risk aneurysms that may enlarge and rupture. Preclinical studies reported the feasibility of detecting macrophages by MRI enhanced with iron nanoparticles in experimental AAA [40]. A recent clinical study demonstrated that a similar approach with MRI and USPIO (ferumoxtran) can visualize macrophages in AAA and predict future growth [41]. FDG-PET/CT has been used to image macrophages in aortic or cervical artery dissection in patients [42, 43]. Excessive accumulation of activated microglia and macrophages after acute myocardial infarction may worsen tissue injuries. An interesting study by Lee et al. demonstrated that PET/MR imaging involving FDG-PET combined with delayed enhancement MRI after Gd-DTPA administration detected macrophage accumulation in the remote zone in infarcted hearts. Using MRI enhanced with USPIO (ferumoxytol), a clinical study showed the feasibility of visualizing macrophages after acute myocardial infarction [44]. Schroeter et al. used PET with a double tracer strategy involving 11C-PK11195 and FDG to image activated microglia and macrophages in peri-infarct zones after ischemic stroke [45].
3.9 Macrophage Imaging in Fat Tissue
Recent studies suggest that fat tissue inflammation causes insulin resistance and plays important roles in the pathogenesis of metabolic syndrome [46]. Metabolic overload induces adipocyte enlargement, oxidative stress, and production of adipocytokines. These responses cause macrophage-mediated inflammation and a vicious cycle of chronic inflammation. Metabolic syndrome is recognized as one of the risk factors for atherosclerotic vascular diseases. Visualization of fat tissue inflammation may thus be useful to identify high-risk patients. In ob/ob and high-fat diet-induced obese mice, real-time in vivo imaging system visualized dynamic interaction between macrophage and other types of cells in fat tissues [47].
3.10 Future Perspectives
All cardiovascular diseases mentioned in this article are global health threats. We have summarized the status of preclinical and clinical development of molecular imaging of macrophages targeting such devastating diseases. In the last several decades, tremendous efforts have used histopathology, molecular and cell biology, and animal experiments to explore the mechanisms by which inflammation promotes cardiovascular disorders. Effective therapies for these diseases remain limited. Macrophage imaging, particularly in humans, would provide new insight into the disease mechanisms and the development of new therapies. Molecular imaging, particularly by noninvasive modalities, should help to identify subclinical lesions rich in activated macrophages and initiate an intense anti-inflammatory therapy as an example of personalized medicine. In addition, macrophage imaging may be used in the near future to monitor changes in vascular inflammation during clinical trials for new therapies, contributing to establishing more cost-effective and shorter clinical drug development. Whether imaging as a biomarker can add additional benefits to the current strategies using conventional risk markers, however, remains uncertain. In addition, each modality has advantages and disadvantages. Thus, the combined use of multiple modalities may play a major role in clinical imaging [48]. Furthermore, as we have witnessed in the last decade, active multidisciplinary research facilitates technological advancements of molecular imaging. More dynamic cross-sector collaboration may speed clinical translation of new technologies and preclinical findings.
References
1.
Libby P, Aikawa M. Stabilization of atherosclerotic plaques: new mechanisms and clinical targets. Nat Med. 2002;8(11):1257–62. doi:10.1038/nm1102-1257.PubMedCrossRef
2.
Deguchi J, Aikawa M, Tung CH, Aikawa E, Kim DE, Ntziachristos V, Weissleder R, Libby P. Inflammation in atherosclerosis: visualizing matrix metalloproteinase action in macrophages in vivo. Circulation. 2006;114(1):55–62. doi:10.1161/CIRCULATIONAHA.106.619056.PubMedCrossRef
3.
Sukhova GK, Shi GP, Simon DI, Chapman HA, Libby P. Expression of the elastolytic cathepsins S and K in human atheroma and regulation of their production in smooth muscle cells. J Clin Invest. 1998;102(3):576–83. doi:10.1172/JCI181.PubMedCentralPubMedCrossRef
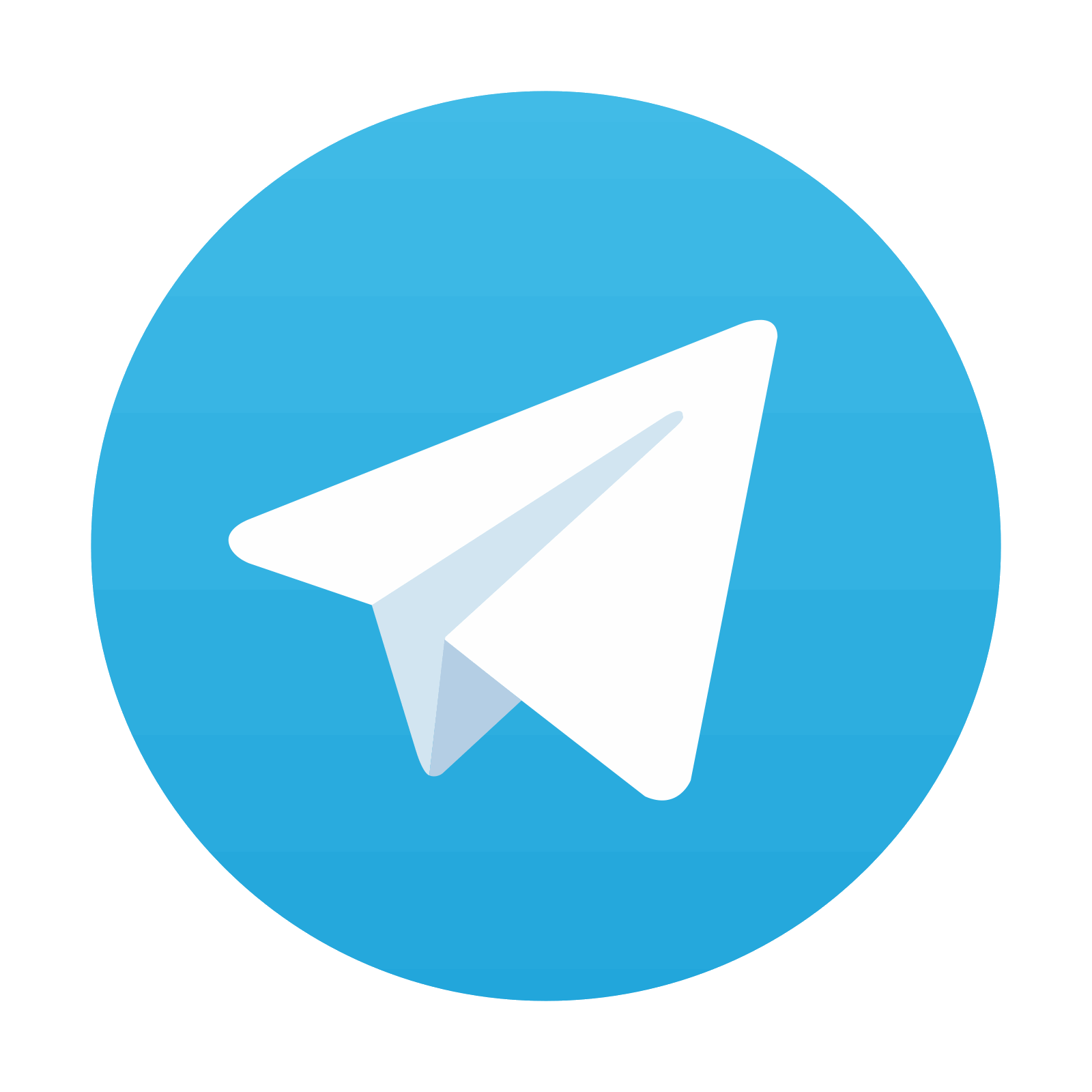
Stay updated, free articles. Join our Telegram channel
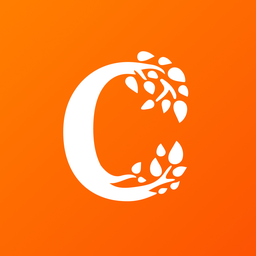
Full access? Get Clinical Tree
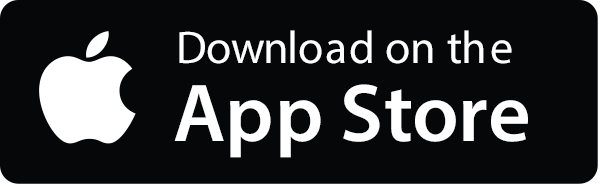
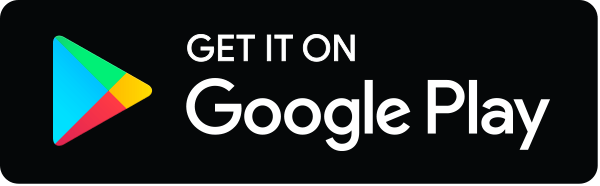