Fig. 13.1
Schematic overview of plaque development. Starting from the upper left panel (I) with a healthy vessel wall, in counterclockwise direction, each panel shows the major molecular and cellular processes during different stages of plaque progression
The degree of luminal stenosis is currently regarded as the key indicator for treatment [2]. Highly stenosed vessels, however, often have thick fibrous caps, making them stable and less prone to rupture and thrombotic events [4]. During plaque formation outward remodeling may occur, leading to plaque progression with little effect on lumen diameter [3]. Although stenosed vessels may cause ischemia, the majority of acute clinical events are initiated by rupture of plaques without flow-limiting stenosis [3].
13.1.3 Animal Models of Atherosclerosis
Genetically modified mice are commonly used as a model of atherosclerosis. Of these the apolipoprotein E-knockout (ApoE −/−) mouse is the most widely used, followed by the LDL-receptor knockout (LDL-R −/−) mouse [6]. ApoE −/− mice have elevated cholesterol levels and spontaneously develop atherosclerosis throughout the major arteries. Often this process is accelerated by a high-fat/high-cholesterol diet. The LDL-R −/− mouse on a regular diet more slowly develops atherosclerosis than the ApoE −/−mouse. Also in this model, plaque development is significantly accelerated by a high-fat/high-cholesterol diet. Both mouse models eventually develop advanced plaques with a lipid-rich necrotic core, a fibrous cap, calcifications, and active inflammation. In contrast to humans, plaques in these mice will not rupture spontaneously. The Watanabe heritable hyperlipidemic (WHHL) rabbit and the New Zealand white (NZW) rabbit are also commonly used in atherosclerosis research [7]. WHHL rabbits have a natural mutation in the LDL-R gene and form unprompted atherosclerotic lesions, while other rabbit models such as the NZW need an atherogenic diet to initiate plaque formation. Additionally, atherosclerosis can be triggered by mechanical injuring of the endothelium, e.g., by balloon denudation. Models of plaque rupture may be generated by mechanical disruption of the atherosclerotic plaque. A large animal model of atherosclerosis is the swine. The pig has characteristics that are more similar to humans than small animal models, not only because of a similar body size. They also possess similar dietary preferences, metabolism, and a similar predisposition for developing atherosclerosis [7]. Nevertheless, small animals are easier to house and breed, are easier to handle, and, because of the smaller body size, require lower amounts of therapeutic compound or contrast agent. This makes the use of small animal models less costly. A difficulty of small animals may be to gather enough blood or tissue for ex vivo analysis.
13.1.4 Magnetic Resonance Imaging
Protons (1H) possess a property called spin, associated with a nuclear magnetic moment, which aligns in parallel or antiparallel direction to an applied magnetic field. Due to a small imbalance in the number of parallel and antiparallel aligned proton spins, a net magnetization oriented parallel to the magnetic field will arise. In MRI, an electromagnetic induction signal is generated from this net magnetization by radiofrequency (RF) excitation. After excitation, the proton magnetization will relax back to equilibrium. The rate of this process is governed by the longitudinal or spin-lattice (T1) and transversal or spin-spin (T2) relaxation times. Relaxation rates are defined as R1 = 1/T1 and R2 = 1/T2. T2 may be shortened by local field inhomogeneities, and the effective spin-spin relaxation time is then called T2*. Specifically designed RF pulse sequences are used to generate images with contrast between tissues based on differences in proton density, T1, T2, or T2*. MR contrast agents interact with water protons in their environment and lower the T1 and T2 relaxation times. This ability of a contrast agent to lower T1 and T2 is expressed in its relaxivities r1 and r2, in units of per millimolar per second (mM−1 s−1). The ratio r2/r1 determines whether an agent is most efficient as a T1 or T2 contrast agent. T1 contrast agents are typically based on the lanthanide gadolinium in the form of the Gd3+ ion and are characterized by a low r2/r1 ratio. By shortening the T1 of surrounding water protons, a local signal increase in the T1-weighted MR image is observed, and therefore these agents are also known as positive contrast agents. Contrast agents which shorten T2 and T2*, such as iron oxide nanoparticles, have high r2/r1 ratios and lead to signal decrease or darkening of T2– or T2*-weighted MR images. Hence, they are called negative contrast agents. In molecular MRI, contrast agents are exploited to report on molecular and cellular processes. Typically, this involves binding to a specific molecular marker by a compatible ligand, which is attached to a carrier containing the contrast agent. This concept is also used to enhance detection sensitivity by increasing the number of contrast agent moieties per carrier and thus ultimately per successfully targeted biomarker.
An essential MRI parameter is the field strength. In the clinical setting, 1.5 T and 3 T are most commonly used, but the number of 7 T scanners utilized for clinical research is steadily growing. Dedicated preclinical small animal scanners usually have a higher magnetic field strength ranging from 4.7 T to 17.6 T. High-field MRI scanners benefit from a higher signal-to-noise ratio (SNR) and higher spatial resolution. However, contrast agent relaxivities are field strength dependent. The r1 of Gd-containing agents strongly decreases at high field strength [8]. In contrast, iron oxide nanoparticles may benefit from higher field strengths because of increasing r2 and r2/r1 ratio.
Not all MRI applications require the use of a contrast agent, though. Figure 13.2 shows some examples of endogenous contrast MRI relevant to atherosclerosis imaging. Time-of-flight (TOF) MRI exploits the inflow of blood for luminal imaging by saturating all static tissues and can thus be used to detect arterial stenosis (Fig. 13.2a). Multi-contrast MRI protocols (TOF, T1-weighted, T2-weighted, proton density-weighted, diffusion-weighted) enable identification of various plaque features such as fibrous matrix, necrotic core, calcification, and hemorrhage or thrombus. Classification of these features is based on their hypo-, hyper- or isointense appearance on the various weighted images and allows for coarse plaque staging (Fig. 13.2B) [10]. Calcifications appear as regions of hypointense signal on these traditional weightings due to a low water content and low T2, and detection is thus based on the absence of signal making unambiguous detection of calcification difficult. Recent developments in ultrashort echo time (UTE) MRI allow for hyperintense imaging of tissue components with very low T2 values, enabling assessment of calcification density and providing a valuable addition to the multi-contrast MRI protocols [12–14]. Fresh thrombi and intra-plaque hemorrhage appear as hyperintense areas on T1-weighted images because they contain methemoglobin which shortens T1 (Fig. 13.2C) [11, 15]. These are examples of the clinical application of non-contrast-enhanced MRI, and the preclinical use of such methods has also been explored [16]. Another example of non-contrast-enhanced MRI is the use of flow-sensitive MRI techniques to distinguish areas with low shear stress [17], which is believed to be an early inducer of atherosclerosis [18]. Furthermore, nontargeted dynamic contrast-enhanced (DCE) imaging approaches are used for evaluating endothelial permeability [19] or neovascularization [20]. DCE-MRI comprises the measurement of T1-weighted images with a high temporal resolution during the intravenous injection of a low molecular weight Gd-based contrast agent. Microvessel density and permeability may be derived by pharmacokinetic modeling of dynamic signal enhancement. Low molecular weight Gd-based contrast agents are clinically available, and DCE-MRI has therefore become an important method in clinical research. Typical doses of low molecular weight Gd-based contrast agents are in the order of 0.1 mmol per kilogram bodyweight.
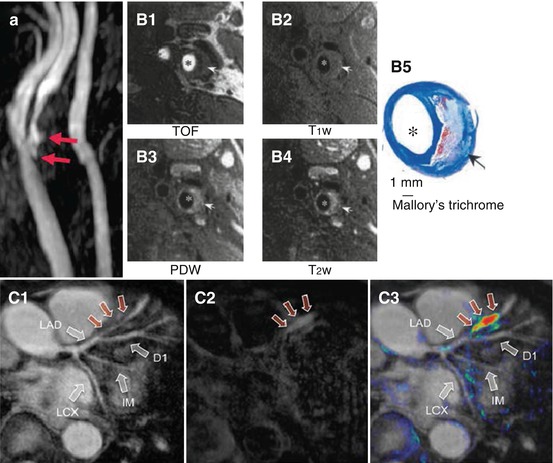
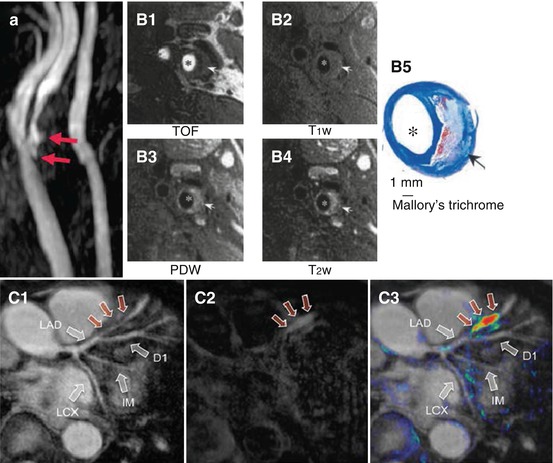
Fig. 13.2
Three types of non-contrast-enhanced MRI, which are relevant to atherosclerosis imaging. (a) Time-of-flight (TOF) MR angiogram of a patient with stenosis in the right internal carotid artery. Arrows point at plaque ulceration. (B1) TOF, (B2) T1-weighted, (B3) proton density-weighted (PDW), and (B4) T2-weighted MR images of a carotid artery atherosclerotic plaque in a patient scheduled for endarterectomy compared to (B5) Mallory’s trichrome histology showing a lipid-rich necrotic core (arrow) and lumen. (C1) TOF, (C2) T1-weighted, and (C3) false-color overlay of T1 signal intensity on TOF MR images show evidence of a thrombus in the left anterior descending (LAD) coronary artery of a patient (red arrows). LCX left circumflex artery, IM ramus intermedius (Images were adapted with permission from (a) Josephs et al. [9], (B) Cai et al. [10], and (C) Jansen et al. [11])
Next, major biological characteristics of atherosclerotic plaques are discussed in relation to the relevant biomarkers and molecular imaging strategies. These characteristics include inflammation, the lipid core, the fibrous cap, thrombus formation, intra-plaque hemorrhage, apoptosis, and neovascularization.
13.2 Inflammation
Plaque inflammation plays an important role in the development and progression of atherosclerosis, and active inflammation is believed to be one of the major hallmarks of high rupture risk [5]. Inflammation is characterized by infiltration of macrophages and T-cells. Macrophages have the tendency to phagocytize foreign bodies – a trait which has been exploited for passive targeting of contrast materials. Furthermore, active targeting strategies have been developed for plaque inflammation imaging.
13.2.1 Active Targeting
Active targeting can be defined by the use of a ligand to achieve selective binding of the contrast agent to a molecular target of interest. For imaging of plaque inflammation several targets are available. The first stage of inflammation is the recruitment of blood-borne pro-inflammatory cells by the expression of adhesion molecules on endothelial cells. These cell-surface receptors can therefore be exploited for molecular imaging during the first stage of inflammation. Examples include VCAM-1 targeting with ultrasmall superparamagnetic iron oxide particles (USPIO) [21, 22] and Gd-based contrast agents [23], ICAM-1 targeting with micron-sized particles of iron oxide (MPIO) and Gd-liposomes [24], as well as CD44-specific imaging with superparamagnetic iron oxide nanoparticles (SPION) [25]. McAteer et al. showed that simultaneous targeting of MPIO to two types of adhesion molecules, VCAM-1 and P-selectin, yields greater binding affinity in vitro and more effective and more rapid homing to activated endothelium in vivo (Fig. 13.3) [26]. This latter aspect of targeting kinetics is important for clinical application, where injection of the agent and imaging should be feasible within a single clinical examination for reasons of efficiency.
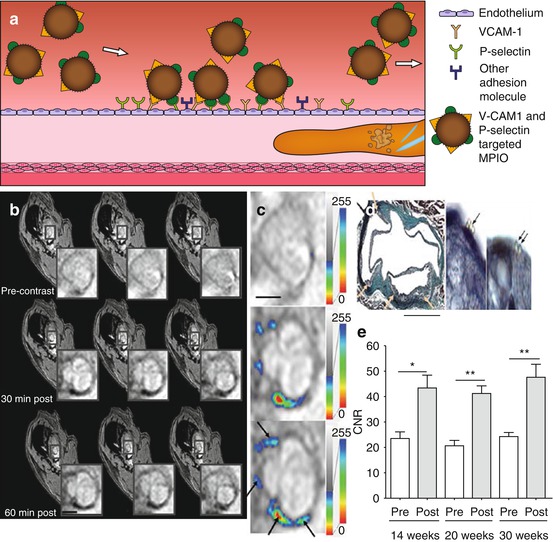
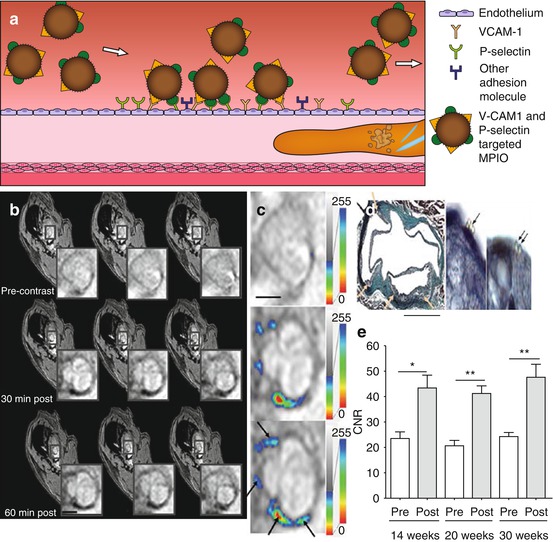
Fig. 13.3
(a) Targeted inflammation imaging, in which micron-sized particles of iron oxide (MPIO) conjugated with ligands for adhesion molecules VCAM-1 and P-selectin home rapidly to activated endothelium. (b) T2*-weighted images of the aortic root of ApoE −/− mice after 14 weeks on regular chow diet show signal decrease at 30 and 60 min postinjection compared to preinjection. (c) T2* signal intensity maps show the distribution of MPIO indicated by false color-coded overlay. (d) Histology (Masson trichrome and elastin stain) confirms that MPIO adhesion is confined to atherosclerotic foam cell lesions. (e) Contrast-to-noise ratio (CNR) of atherosclerotic lesions was significantly increased after injection of dual-targeted MPIO with no significant difference between time points. (b–d) Scale bars = 1 mm (Images (b–e) were adapted with permission from McAteer et al. [26])
The location of the target restricts or dictates the useable size of the targeted contrast agent. Large particles such as MPIO and Gd-liposomes have a high relaxivity per particle, making them ideal contrast agents for intravascular targets like adhesion molecules. Since larger particles have more difficulty to extravasate, smaller contrast agents, such as USPIO, Gd-micelles, and low molecular weight Gd-based contrast agents, are more suitable for intra-plaque targeting. Apart from extravasation, retention of the agent in the plaque should be considered. Van Bochove et al. compared the passive accumulation of three differently sized Gd-based contrast agents, i.e., Gd-HP-DO3A, micelles, and liposomes, in mouse atherosclerotic carotid plaques [27]. It was concluded that Gd-micelles of ~15 nm are able to permeate the plaque abundantly, whereas Gd-liposomes with a diameter of ~125 nm remain mostly intravascular and therefore would be more suitable for intravascular targets.
Other strategies for plaque inflammation target the macrophages and foam cells directly. Proteins, such as scavenger receptors, expressed on the cell surface have been targeted using USPIO [28], Gd-micelles [29, 30], and Gd-metallofullerene-containing liposomes [31]. LOX-1, which is involved in oxLDL binding and expressed on macrophages and other cell types involved in inflammation, has been targeted with USPIO [32]. The most common way to directly target plaque-associated macrophages is, however, by passive targeting exploiting the natural phagocytic property of this inflammatory cell type.
13.2.2 Passive Targeting
Macrophages readily phagocytize foreign bodies, and this behavior is widely exploited for passive targeting of MRI contrast materials. Nontargeted USPIOs were shown to accumulate in macrophage-rich plaques upon intravenous injection in rabbits [33] as well as in symptomatic patients [34]. Experimental studies in ApoE −/− mice showed the ability of the technique to image both age-related plaque progression and the effects of treatment with anti-inflammatory drugs (Fig. 13.4) [35–37]. Passive macrophage targeting using USPIOs has thus become a useful preclinical tool for readout of the plaque inflammatory status. We believe that standardization of the technique should be improved to allow more direct comparisons between studies performed in different research centers and on different MR systems. This will allow for better comparison of different therapies that aim for reduction of atherosclerosis burden via reduction of inflammation. This requires standardization of the USPIO preparations, dose, animal models, and acquisition method. With respect to the latter factor, standardization can be improved by the use of quantitative imaging, specifically T2* mapping [36, 37], T2 mapping [38, 39], or susceptibility gradient mapping (SGM) [35] for the evaluation of iron oxide accumulation. Such techniques in principle allow for a quantification of iron content in the plaque, which should, however, be interpreted with care since, although predominantly macrophage associated, iron content is not a direct measure of the number of macrophages. Although the quantified imaging readout is a result of the cumulative effect of macrophage content, activity, plaque permeability, and contrast material pharmacokinetics, collectively it may still provide a quantifiable readout of overall inflammatory status that correlates with plaque progression and treatment response. USPIO inflammation imaging shows clinical potential. Gillard et al. have demonstrated feasibility in long-term follow-up studies [40], and although USPIO-induced MR signal changes were not proven to be predictive for clinical events, this was most likely due to a lack of statistical power [41]. USPIO-enhanced T2* quantification was demonstrated for treatment monitoring in a clinical setting [42]. It was shown that preinjection MRI scans were not needed for assessing differences in USPIO uptake. Most of the early studies have been performed with Sinerem (ferumoxtran-10), a dextran-coated USPIO of approximately 30 nm. However, currently Sinerem is not commercially available anymore, as production has been discontinued by the manufacturer. Ferumoxytol, a ~30 nm carbohydrate-coated USPIO, was found to induce larger signal changes than Sinerem in preclinical studies [43, 44]. Ferumoxytol is an FDA-approved supplement for treatment of iron deficiency but also serves as an MRI contrast agent [45]. Ferumoxytol is investigated for imaging the inflammatory response in patients with acute myocardial infarction [46] and is currently evaluated in a clinical trial for monitoring inflammation in carotid atherosclerosis [47].
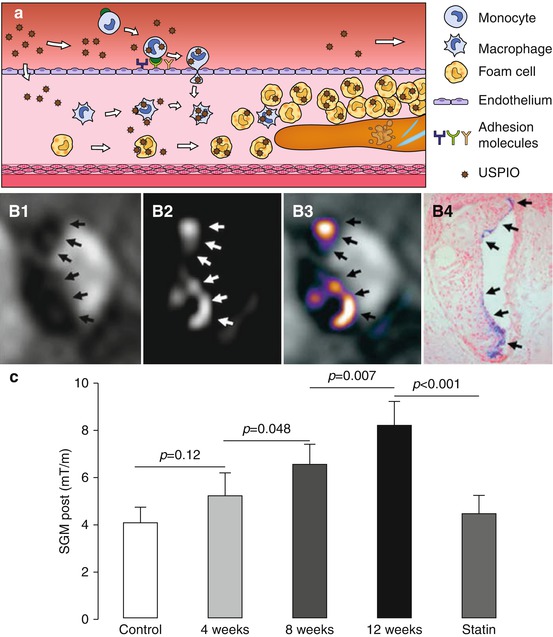
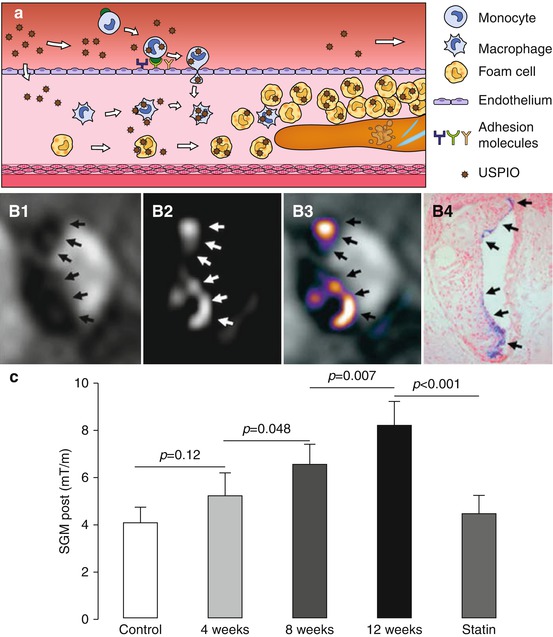
Fig. 13.4
(a) Passive targeting of plaque inflammation with ultrasmall superparamagnetic iron oxide (USPIO) nanoparticles. USPIO are taken up via phagocytosis by blood monocytes, plaque macrophages, and foam cells. (b) In vivo imaging of plaque in the brachiocephalic artery of ApoE −/− mice on a 12 week high-fat diet 48 h post USPIO injection: (B1) dark spots on T2*-weighted image, (B2) bright signal on susceptibility gradient mapping (SGM), (B3) SGM overlay, and (B4) Perls’ iron stain histology. (c) SGM values increased with plaque progression and decreased to control levels in the statin-treated group (Images (b, c) were adapted with permission from Makowski et al. [35])
Nontargeted perfluorocarbon (PFC) nanoparticles are also phagocytized by macrophages and thus can be exploited for molecular imaging of inflammation, with the additional advantage that PFC can be imaged by 19F MRI separately from the proton anatomical image. PFC inflammation imaging has been explored in various pathologies [48, 49] and holds promise for plaque inflammation as well. 19F MRI of PFC-containing nanoparticles has several advantages over traditional (T1 and T2) proton-detected contrast agents. The 19F MR signal is directly proportional to the PFC concentration, and background fluorine signal is absent resulting in a high detection specificity. Furthermore, some PFC-containing nanoparticles suitable for MRI purposes are FDA approved as blood substitutes [50]. Nevertheless, detection of low numbers of macrophages using the fluorine approach may remain challenging because of sensitivity issues [51, 52].
13.3 Lipids
Atherosclerotic plaques with a large core containing lipids and cellular debris are considered at risk of rupture. Plaque-borne lipids are involved in the early stages of plaque formation, when LDL enters the intima where it is oxidized and taken up by macrophages to form foam cells. This process continues throughout plaque progression, and a large plaque core is a strong predictor of rupture. Improved visualization of the lipid-rich core was achieved using lipophilic contrast agents such as Gd-based gadofluorine M, which accumulates in lipid-rich areas of the plaque [53]. USPIO [54, 55] and Gd-micelles [56] were targeted to oxLDL. Alternatively, manganese (Mn(II))-containing micelles were used [56]. While in the blood, these micelles have low relaxivity; once bound to oxLDL, however, the micelles become internalized by macrophages and release their manganese payload in the intracellular compartment, resulting in a strongly increased relaxivity. This renders the observed signal enhancement on T1-weighted MRI specific to macrophage and foam cell phagocytic activity.
Native or synthetically produced LDL and HDL can be modified to include MRI contrast agent facilitating imaging of plaque uptake. Injection of Gd-containing LDL-like nanoparticles led to increased signal intensity in atherosclerotic plaque by macrophage uptake [57]. HDL is responsible for reverse cholesterol transport from macrophages and thus may serve both imaging as well as therapeutic purposes [58]. For that purpose, several versions of HDL-like particles were designed for molecular imaging with various imaging modalities [58]. Recently, it was revealed that the effective plaque enhancement from Gd-containing HDL-like particles is mediated by transfer of Gd-phospholipids to LDL which in turn are taken up by macrophages [59]. HDL can be designed to incorporate contrast-generating nanoparticles in the HDL core, including USPIO, for T2-weighted MRI, and gold nanoparticles for CT [60]. The delivery method of these particles is important. Intraperitoneal injection of USPIO-containing HDL-like particles yields a constant blood concentration over several hours allowing for higher uptake compared to intravenous injection [61]. In vivo particle accumulation in the plaque was not sufficient to induce significant signal changes in T2*-weighted MRI. Nevertheless, ex vivo T2* mapping showed a significant difference between native and postinjection T2* relaxation times (Fig. 13.5) [61]. Advanced T2 or T2* mapping methods may facilitate more robust in vivo detection and quantification of nanoparticle uptake [38, 39].
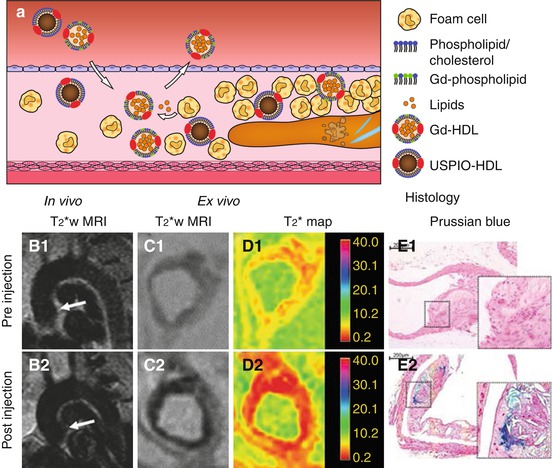
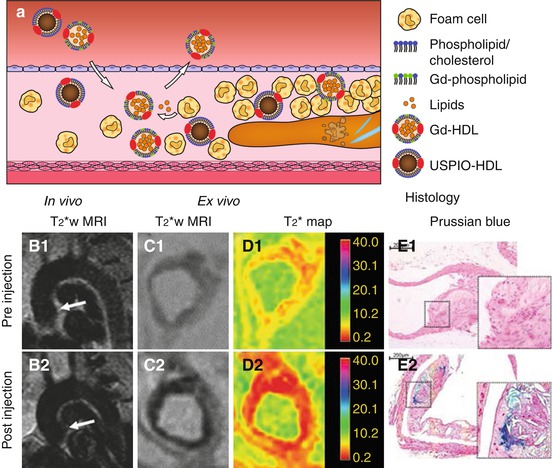
Fig. 13.5
(a) High-density lipoprotein (HDL) homes to sites with high macrophage/foam cell content and is involved in reverse cholesterol transport. HDL-like particles can be functionalized, e.g., with Gd-phospholipids in the phospholipid layer or USPIO particles in their core and subsequently can individually be used for molecular MR of plaque inflammation. (b) In vivo T2*-weighted MRI of the aortic arch of ApoE −/− mice (B1) before and (B2) 24 h after USPIO-HDL injection shows signal decrease upon USPIO-HDL uptake. (C1, 2) Ex vivo T2*-weighted MRI shows a similar decrease in signal in the aortic root which was quantified with (D1, 2) T2* mapping. (e) Prussian blue iron staining confirmed the uptake of iron particles in (E2) the plaque of injected mice but not (E1) in non-injected mice (Images (b–e) were adapted with permission from Jung et al. [61])
13.4 Fibrous Cap and ECM Components
A thick fibrous cap is considered a feature of plaque stability. The fibrous cap contains SMC and ECM components such as collagen and elastin. Pro-inflammatory macrophages may excrete proteinases such as matrix metalloproteinases (MMP), breaking down the ECM components, destabilizing the plaque, and increasing the chance of rupture. Both the ECM and the presence of proteinases, such as MMP, therefore have been explored as molecular imaging targets.
The most abundant component of the fibrous cap is collagen. In a mouse model of plaque regression, Gd-containing HDL-like particles functionalized with a collagen-binding peptide (EP3533) were used to image collagen content during the change from a phenotype with many macrophages and little collagen into a phenotype with few macrophages and increased collagen content (Fig. 13.6) [62]. Collagen-functionalized particles generated significant signal enhancement in the regressed phenotype with high collagen content, while their nonfunctionalized counterparts did this in the vulnerable, high macrophage content phenotype. Since both HDL-like particles are equipped with Gd for MRI detection, they provided positive and therefore easier detectable contrast. Because both agents provided similar contrast, concurrent use of both particles, however, was not possible in a single subject. The choice for a nonfunctionalized or a functionalized particle is dependent on the kind of target and application. For monitoring therapy aimed at increasing collagen content, collagen-binding HDL-like particles will likely be the preferred choice. Gd-micelles functionalized with the collagen-binding protein CNA35 are another promising contrast agent for imaging of collagen, as demonstrated in an ApoE −/− mouse model of relatively collagen-rich and collagen-poor carotid atherosclerosis (Fig. 13.7) [63]. Another important target of the ECM in atherosclerosis is elastin. A low molecular weight Gd-containing contrast agent, the elastin-specific MR contrast agent (ESMA), enabled assessment of plaque progression and statin-induced regression in a mouse model (Fig. 13.8b, c) [64]. ESMA was subsequently used in swine models for detection of vascular remodeling after coronary wall injury [66] and was shown to facilitate excellent three-dimensional aortic vessel wall imaging (Fig. 13.8d1–2) [65].
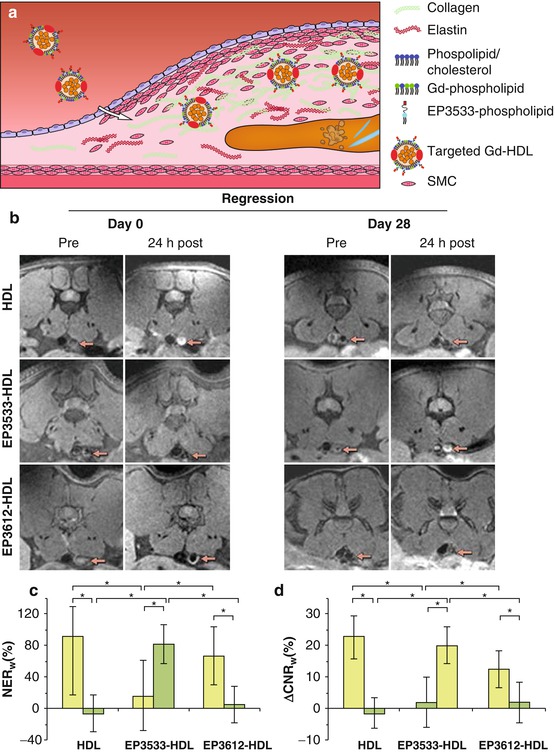
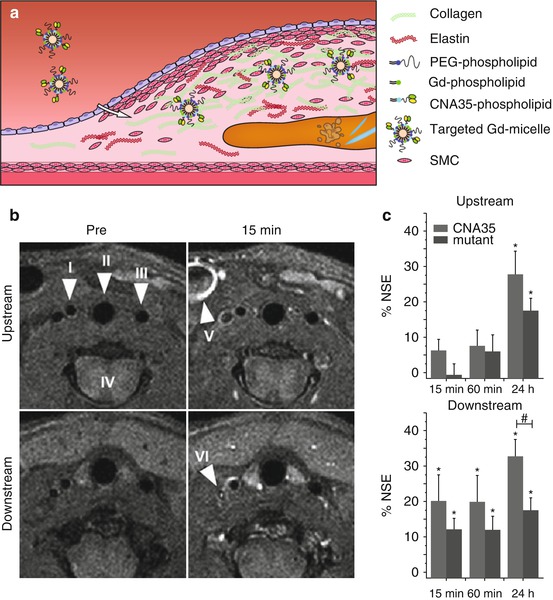
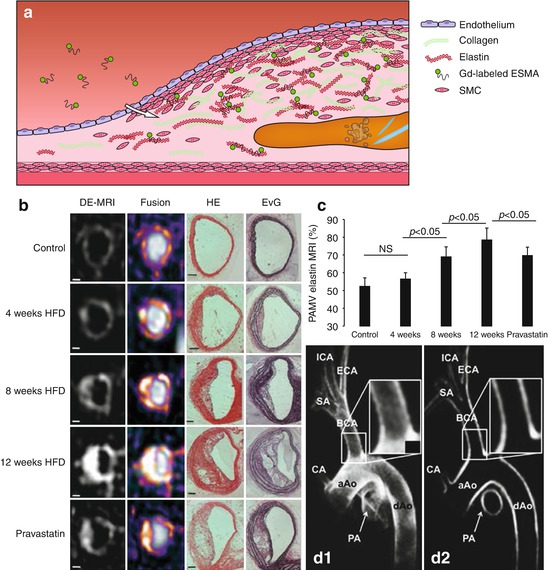
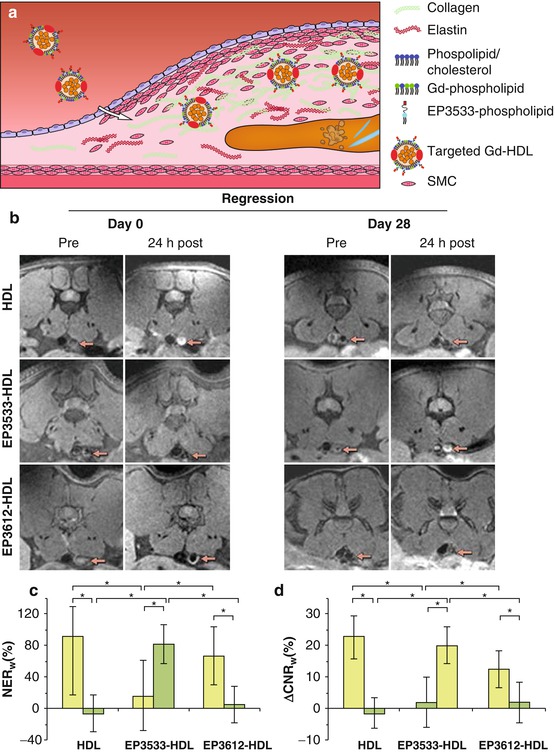
Fig. 13.6
(a) Gd-HDL particles functionalized with the EP3533 peptide bind to collagen in the atherosclerotic plaque. (b) MR images before and 24 h after injection of nontargeted HDL-like particles, collagen-targeted EP3533-HDL, and EP3612-HDL as a nonfunctional control at day 0 and day 28 of plaque regression in the abdominal aorta in a mice (arrows point to the aorta). (c) Normalized enhancement ratio (NER w ) and (d) the difference between pre- and postinjection contrast-to-noise ratios (ΔCNR w ) of the aortic wall show a significant increase for EP3533-HDL and significant decrease for HDL and EP3612-HDL between day 28 (green bars) and day 0 (yellow bars) (Images (b–d) were adapted with permission from Chen et al. [62])
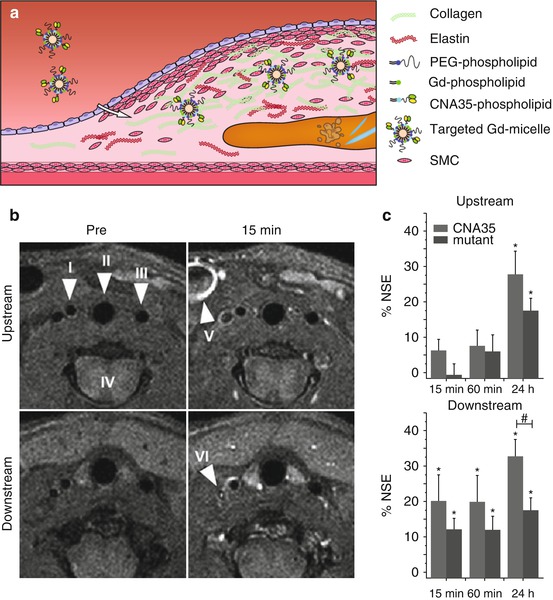
Fig. 13.7
(a) Gd-micelles functionalized with CNA35 protein and protected from detection by the immune system with polyethylene glycol (PEG)-phospholipids bind to collagen in the atherosclerotic plaque. (b, c) In ApoE −/− mice a cast was placed in the right carotid artery, leading to induction of plaques with a difference in collagen content upstream and downstream (more collagen) from the cast. (b) T1-weighted imaging shows enhancement of the plaque richer in collagen already 15 min after injection of CNA35 micelles. Arrowheads indicate the right carotid artery (I), trachea (II), left carotid artery (III), spinal cord (IV), external jugular vein (V), and internal jugular vein (VI). (c) The calculated percentage normalized signal enhancement (% NSE) upon CNA35-micelle injection shows significant enhancement (* = p < 0.05) in the downstream collagen-rich plaque at all time points, yet only significantly different compared to mutant (dysfunctional) CNA35 micelles (# = p < 0.05) at 24 h after injection. In the upstream plaque, significant enhancement is only reached after 24 h (Images (b, c) were adapted with permission from van Bochove et al. [63])
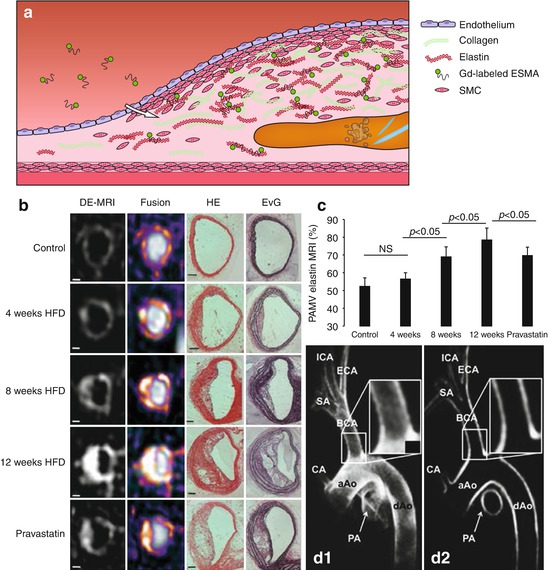
Fig. 13.8
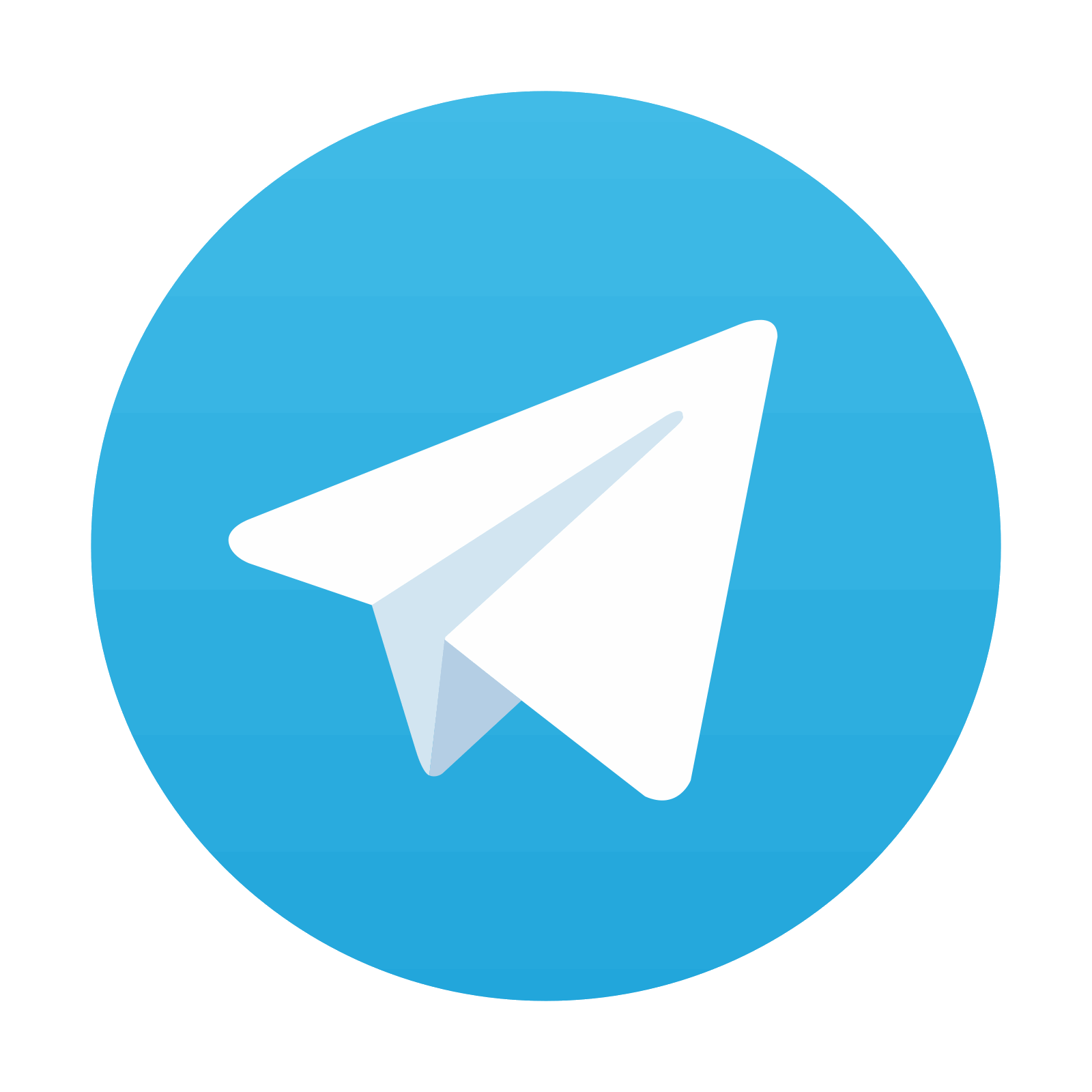
(a) The elastin-specific MR contrast agent (ESMA) consists of a small peptide conjugated to a Gd-chelate and can bind to elastin in the atherosclerotic plaque. (b) Delayed-enhancement (DE) MRI and fusion images with DE-MRI overlaid on time-of-flight (TOF) images show that ESMA-induced MRI signal increases with plaque progression in the brachiocephalic artery of ApoE −/− mice on a high-fat diet, except for pravastatin-treated animals. Plaque progression and increased elastin content were confirmed with histology using hematoxylin and eosin (HE) and Elastica van Gieson (EvG) staining. Scale bars 250 μm. (c) The MR images allow estimation of the percentage atheroma/media volume (PAMV) which increases with plaque progression, but significantly less in the pravastatin-treated group. (d) Excellent 3D vessel wall imaging with ESMA was demonstrated in the porcine aortic arch: (D1) maximum intensity projection and (D2) multi-planar reconstructed images 90–100 min after injection. aAo ascending aorta, BCA brachiocephalic artery, CA proximal coronary artery, dAo descending aorta, ECA external carotid artery, ICA internal carotid artery, PA pulmonary artery, SA subclavian artery (Images (b, c) were adapted with permission from Makowski et al. [64] (d) [65])
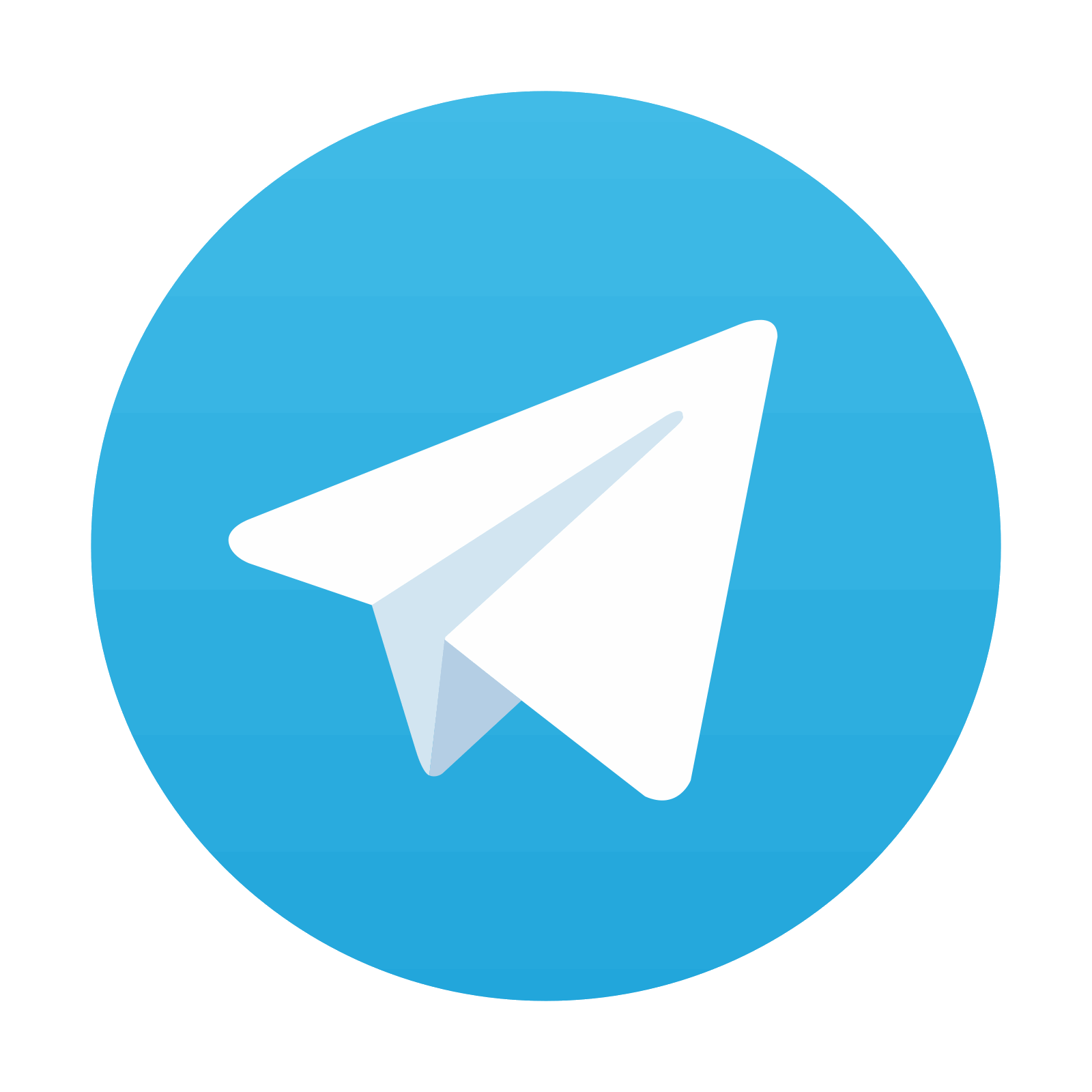
Stay updated, free articles. Join our Telegram channel
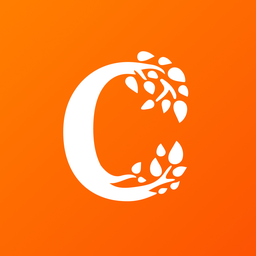
Full access? Get Clinical Tree
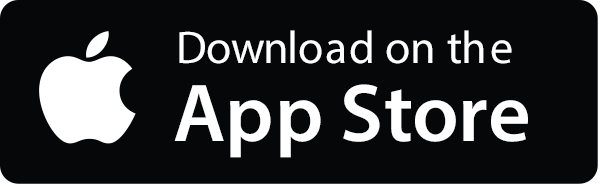
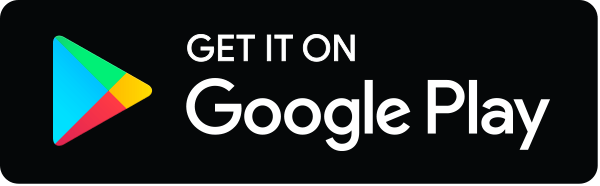
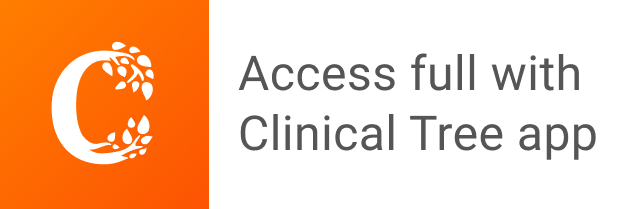