Because articular cartilage is avascular and has no intrinsic capacity to heal itself, physical damage to cartilage poses a serious clinical problem for orthopedic surgeons and rheumatologists. No medication exists to treat or reconstitute physical defects in articular cartilage, and pharmacotherapy is limited to pain control. Developments in the field of articular cartilage repair include microfracture, osteochondral autografting, osteochondral allografting, repair with synthetic resorbable plugs, and autologous chondrocyte implantation. MR imaging techniques have the potential to allow in vivo monitoring of the collagen and proteoglycan content of cartilage repair tissue and may provide useful additional metrics of cartilage repair tissue quality.
Articular cartilage injury poses a serious problem for orthopedic surgeons. The associated pain and physical disability can have career-ending consequences in athletes and can restrict the ability to perform activities of daily living in any individual. In two separate studies, consisting of 31,156 and 1000 patients undergoing knee arthroscopy, cartilage lesions were found in greater than 60% of all patients, and approximately 5% of these lesions were classified as deep partial-thickness or full-thickness defects in patients less than 40 years of age.
Because articular cartilage is avascular, the transport of inflammatory mediators and cells to the site of tissue injury is limited; thus, cartilage has no intrinsic capacity to heal itself. Over the past two decades, there have been many exciting developments in the field of articular cartilage restoration. Patients who were previously offered only symptomatic relief now have a variety of treatment options, including microfracture, osteochondral autografting and allografting, restoration with resorbable synthetic scaffolds, and autologous chondrocyte implantation (ACI) (including matrix-assisted ACI [MACI]). This article reviews the different types of cartilage repair procedures and discusses their assessment using imaging.
Brief overview of MR imaging of cartilage
Many MR imaging sequences are currently used to evaluate cartilage morphology. Typical sequences include fat-suppressed, 3-D, gradient-echo techniques and fast spin-echo techniques with and without fat suppression. These sequences have been extensively used to evaluate articular cartilage and have reported sensitivities and specificities for the detection of cartilage lesions close to or greater than 90% in some studies.
There has also been great interest recently in the use of MR imaging techniques to evaluate the biochemical composition of the cartilage matrix. These techniques include T2 mapping, delayed gadolinium-enhanced MR imaging of cartilage (dGEMRIC), T1rho mapping, and sodium MR imaging. T2 mapping reflects cartilage collagen content and hydration status, whereas dGEMRIC, T1rho mapping, and sodium MR imaging are all markers of cartilage proteoglycan content. Although these biochemical imaging techniques are less widely available, they may provide useful additional information regarding the status of the cartilage repair procedure.
Finally, with the development of ultra–high-field MR imaging scanners (7 T and above), there is the potential to improve on scanning techniques performed at standard clinical field strength (1.5 to 3 T). Because of the greater intrinsic signal-to-noise ratio available at ultra–high field (signal-to-noise ratio scales approximately with the magnitude of the magnetic field), images can be obtained with increased spatial resolution or decreased scan time. Ultra–high field also facilitates the performance of biochemical imaging techniques, such as sodium MR imaging, which is limited by low signal-to-noise ratio at standard clinical field strength.
Parameters assessed in MR imaging of cartilage repair
Multiple parameters should be assessed in MR imaging examinations of cartilage repair. In one study of patients who underwent either microfracture or ACI, the following MR imaging parameters were evaluated: signal intensity relative to native cartilage; morphology with respect to native cartilage (flush, proud, or depressed); delamination (in the setting of ACI); nature of the interface with the adjacent surface (presence or absence and size of fissures); degree of defect filling; integrity of cartilage on the opposite articular surface; and bony hypertrophy.
Another group has proposed a formal grading system for MR imaging assessment of cartilage repair, magnetic resonance observation of cartilage repair (MOCART). Using fat-suppressed, 3-D, gradient-recalled echo, and fast spin-echo sequences, the investigators propose assessment of the following MR imaging parameters: degree of defect filling, integration to border zone, surface of repair tissue, structure of repair tissue, signal intensity of repair tissue, subchondral lamina, subchondral bone, adhesions, and synovitis. This scoring system was later validated in a 2-year longitudinal study of patients with matrix-assisted chondrocyte implantation, with certain parameters, such as degree of defect filling, structure of repair tissue, change in subchondral bone, and signal intensity of repair tissue, correlating well with clinical scores.
Parameters assessed in MR imaging of cartilage repair
Multiple parameters should be assessed in MR imaging examinations of cartilage repair. In one study of patients who underwent either microfracture or ACI, the following MR imaging parameters were evaluated: signal intensity relative to native cartilage; morphology with respect to native cartilage (flush, proud, or depressed); delamination (in the setting of ACI); nature of the interface with the adjacent surface (presence or absence and size of fissures); degree of defect filling; integrity of cartilage on the opposite articular surface; and bony hypertrophy.
Another group has proposed a formal grading system for MR imaging assessment of cartilage repair, magnetic resonance observation of cartilage repair (MOCART). Using fat-suppressed, 3-D, gradient-recalled echo, and fast spin-echo sequences, the investigators propose assessment of the following MR imaging parameters: degree of defect filling, integration to border zone, surface of repair tissue, structure of repair tissue, signal intensity of repair tissue, subchondral lamina, subchondral bone, adhesions, and synovitis. This scoring system was later validated in a 2-year longitudinal study of patients with matrix-assisted chondrocyte implantation, with certain parameters, such as degree of defect filling, structure of repair tissue, change in subchondral bone, and signal intensity of repair tissue, correlating well with clinical scores.
Types of cartilage repair procedures and appearance on MR imaging
Microfracture
Rationale
Microfracture was introduced by Steadman and colleagues in the early 1980s and was described as a treatment for full-thickness posttraumatic cartilage defects. As opposed to other marrow stimulation techniques, such as drilling, there is essentially no risk for thermal necrosis of surrounding tissue. Through micropenetration of the subchondral bone plate, the cartilage defect is populated with platelets, growth factors, and bone marrow-derived mesenchymal stem cells, which mediate a fibrocartilaginous repair process. Fibrocartilage that forms within the defect is less well organized than normal hyaline articular cartilage and has a higher proportion of type I collagen; as a result, its biomechanical properties are inferior to that of hyaline cartilage.
Indications
Microfracture is a simple, single-stage procedure with relatively low patient morbidity. Microfracture is indicated as a first-line treatment for patients with small full-thickness cartilage defects in either the weight-bearing region of the femorotibial compartment or in an area of contact between the patella and trochlea. Unstable cartilage flaps are another indication for microfracture.
Surgical technique
An arthroscopic awl is used to make multiple holes or microfractures in the exposed subchondral bone plate. When fat droplets are seen coming from the marrow cavity, the appropriate depth of 2 to 4 mm has been reached. Significant functional improvement after microfracture has been documented at a minimum follow-up period of 2 years with the best results observed with good fill grade, low body mass index, and a short duration of preoperative symptoms. The success of the procedure in athletes who perform high-impact sports is associated with lesion size less than 200 mm 2 , preoperative symptoms less than 12 months, and no prior surgical intervention. Although weight bearing is detrimental in the first 8 weeks after surgery, continuous passive motion is a critical component of rehabilitation that may help stimulate chondocyte matrix production and remodel the repair cartilage surface so that it is congruent with the native hyaline cartilage.
Imaging studies
The MR imaging appearance of reparative fibrocartilage after microfracture varies depending on the time after surgery. In general, the reparative fibrocartilage is hypertintense relative to native hyaline cartilage ( Figs. 1 and 2 ). Adverse functional scores after 24 months have been correlated with poor percentage of fill by repair tissue and incomplete peripheral integration. Lack of peripheral integration and thinning of the repair tissue may increase mechanical stress on the reparative fibrocartilage, leading to early cartilage degeneration and functional decline.
Two studies have used T2 mapping to evaluate the status of reparative fibrocartilage induced by microfracture. In both studies, fibrocartilage did not demonstrate the characteristic spatial variation in T2 values that is seen with native hyaline cartilage (higher T2 values near the articular surface and lower T2 values near subchondral bone). In addition, the overall global T2 value was lower for fibrocartilage repair tissue than for native hyaline cartilage.
Fibrocartilage formed after microfracture has also been evaluated using dGEMRIC. In one study, fibrocartilage demonstrated a greater difference between precontrast and postcontrast T1 relaxation time compared with repair tissue formed after MACI ; this suggests a lower glycosaminoglycan content in fibrocartilage compared with other types of cartilage repair tissue.
Osteochondral Autograft Transfer
Rationale
The use of osteochondral autografts was first described by Yamashita and coworkers and later popularized by Bobić (single plugs) and Hangody and colleagues (multiple plugs or mosaicplasty). This technique involves repairing a cartilage defect with one or more small cylindrical osteochondral plugs harvested from a relatively non–weight-bearing portion of the patellofemoral joint or from the margin of the intercondylar notch. Histologic evaluations after osteochondral autograft transfer in animal models reveal consistent survival of transplanted hyaline cartilage, deep matrix integration and formation of a composite layer with native surrounding cartilage, ingrowth of fibrocartilage at the osseous base of the defect, and osseous incorporation of the graft with recipient subchondral bone.
Indications
The main indications for osteochondral autograft transfer are focal cartilage defects measuring 1 to 5 cm 2 , typically due to trauma or osteochondritis dissecans. Under unusual circumstances, defects as large as 8 to 9 cm 2 have been repaired, but for lesions of this size, there is limited donor tissue available. Fifty years is the recommended upper age limit for the procedure. Although osteochondral autografting was initially performed only on the weight-bearing surfaces of the femoral condyles and patellofemoral joints, it can also be used to treat cartilage defects of the talus, tibia, humeral capitellum, and femoral head.
Surgical technique
Osteochondral autografting can be performed arthroscopically or via an open arthrotomy. Patients are informed preoperatively of the potential need to harvest plugs from the contralateral knee, especially when treating larger cartilage defects. After gaining surgical access, the size of the cartilage lesion is assessed, and the number, diameter, and position of graft plugs required to fill the defect are determined. Femoral condylar lesions are filled with plugs from the margins of the medial and lateral femoral condyles above the sulcus terminalis, whereas lesions in the trochlear groove are treated with plugs harvested from the intercondylar notch.
Osteochondral autografting requires 2 weeks of non–weight-bearing during the immediate postoperative period followed by 2 weeks of partial weight bearing. Successful clinical outcomes have been reported by many groups. In a 10-year follow-up study of 831 patients, good to excellent results were reported in 92%, 87%, and 79% of femoral condylar, tibial, and patellofemoral implantations, respectively. In one clinical trial comparing osteochondral autografting with ACI, osteochondral autografting was associated with faster recovery at 6, 12, and 24 months, although both procedures resulted in decreased symptoms at 24 months.
Imaging studies
On MR imaging, the position of the osteochondral plug within the recipient site should be evaluated, and there should be no graft migration. Perigraft edema can be found in greater than 50% of patients at 1 year, and this gradually decreases by 3 years ( Figs. 3 and 4 ). The presence of subchondral cysts or persistent extensive perigraft edema at 3 years may reflect poor osseous incorporation. Rarely, osteonecrosis has been reported as a complication of osteochondral autografting. The thickness and congruity of the articular surface should also be evaluated. Ideally, the thickness of the repair cartilage should be similar to that of the surrounding native hyaline cartilage with a smooth, congruent articular surface ( Fig. 5 ). The deep bone-bone interface may demonstrate an incongruent surface, because the osteochondral plug may have been harvested from a location with a differing cartilage thickness.
Osteochondral Allograft
Rationale
Osteochondral allografting involves the replacement of damaged articular cartilage with mature hyaline cartilage from a suitable donor. The advantages of osteochondral allografts over autografts are (1) the avoidance of morbidity from the autograft harvesting procedure, (2) the ability to repair larger defects because more donor tissue can be harvested, and (3) the ability to harvest tissue from a site matching the exact location of a patient’s cartilage defect, which allows more accurate matching of the size and contour of the graft to the defect.
The rationale for this procedure is that the transplanted hyaline cartilage contains living chondrocytes that are capable of supporting the cartilage matrix within the host indefinitely. The osteochondral allograft is considered immunoprivileged, because cartilage is avascular and chondrocytes are protected from host immune surveillance due to their location within the matrix. Donor cartilage is not matched to recipient HLA type or blood type. Procurement and processing of donor tissue is, however, performed according to American Association of Tissue Banks guidelines, which includes an extensive medical, social, and sexual history of the donor as well as serologic testing for HIV, hepatitis, syphilis, and other blood-borne pathogens. As a result, although fresh osteochondral allografts are necessary to ensure maximum chondrocyte survival, tissue is transplanted no sooner than 14 days and sometimes as late as 40 days after harvesting while tests for viral and bacterial contamination are conducted. Approximately 5 million fresh osteochondral allografts have been transplanted over the past decade, and few documented cases of disease transmission have been reported.
Indications
In the knee, osteochondral allografting has been used for multiple indications, including traumatic or degenerative cartilage lesions, osteochondritis dissecans, osteonecrosis, or salvage of a previous cartilage repair procedure. The size of the cartilage defect can be as large as 15 cm 2 . Osteochondral allografting has also been performed in the ankle and hip for similar indications and when other procedures, such as osteochondral autografting, are not feasible.
Surgical technique
Osteochondral allografting is performed through a mini arthrotomy or standard arthrotomy to expose the cartilage lesion. The remaining articular cartilage and damaged subchondral bone are removed. The allograft plug (10 to 35 mm in diameter) is then removed from the donor tissue using a coring reamer, and the graft is shaped to match the size and depth of the recipient lesion site. The graft is then gently inserted with a tamp or with joint compression during range of motion, with loose grafts fixed with bioabsorbable pins or screws. Eighty-five percent 10-year survivorship of allografts has been reported for posttraumatic defects of the femur. Multiple studies have documented 80% to 84% good to excellent results at 6-year follow-up in patients treated with osteochondral allografts for a variety of conditions.
Imaging studies
For osteochondral allografts, the same features should be evaluated on MR imaging as for osteochondral autografts ( Fig. 6 ). In one animal study comparing osteochondral autografts with allografts, MR imaging revealed no statistically significant differences in the MR imaging appearance of autografts and allografts. The MR imaging features evaluated included graft cartilage signal intensity, appearance of the subchondral plate and articular surface (flush, depressed, proud, or displaced), interface with the adjacent cartilage (smooth, partial-thickness, or full-thickness offset), percentage fill of the defect by thirds, trabecular incorporation of the grafts (complete, partial, or poor), signal of bone graft (fat, edema, or fibrosis), and cartilage T2 relaxation times. In 90% of subjects, the investigators noted a persistent cleft at the interface between the graft and host cartilage and concluded that hyaline cartilage cannot regenerate across a physical defect.
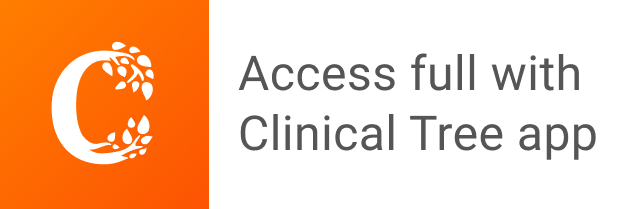