Fig. 25.1
Extradural empyema in a 52- year-old male after resection of a meningioma and cranioplasty. The patient presents fever and frontal region bulging. Postcontrast T1-WI (a) and FLAIR (b) images show some postoperative changes in the frontal lobes and an extra-axial frontal collection with peripheral enhancement. Collection has restricted diffusion (arrows) on DWI (c) and ADC map (d), suggestive of pyogenic collections found in empyema
Patients with medium-size (3–5 cm) and large (>5 cm) postoperative-related intraparenchymal hemorrhage can present with reduced levels of consciousness and focal neurologic deficit or seizures. Extradural hematomas can cause compression of the brain parenchyma. Remote cerebellar hemorrhage can be seen after supratentorial craniotomy and is thought to be related to CSF loss leading to sagging of the cerebellum, with hemorrhagic infarct (Fig. 25.2).
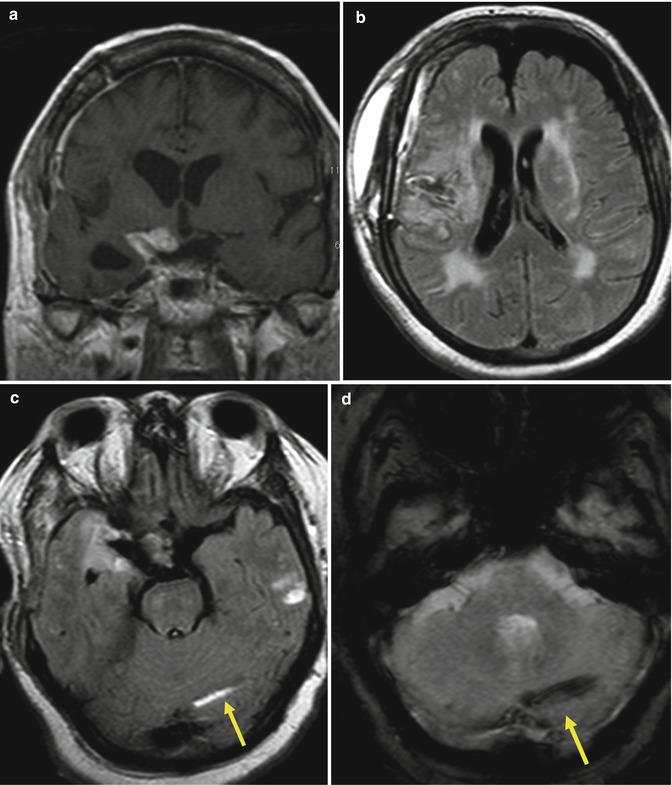
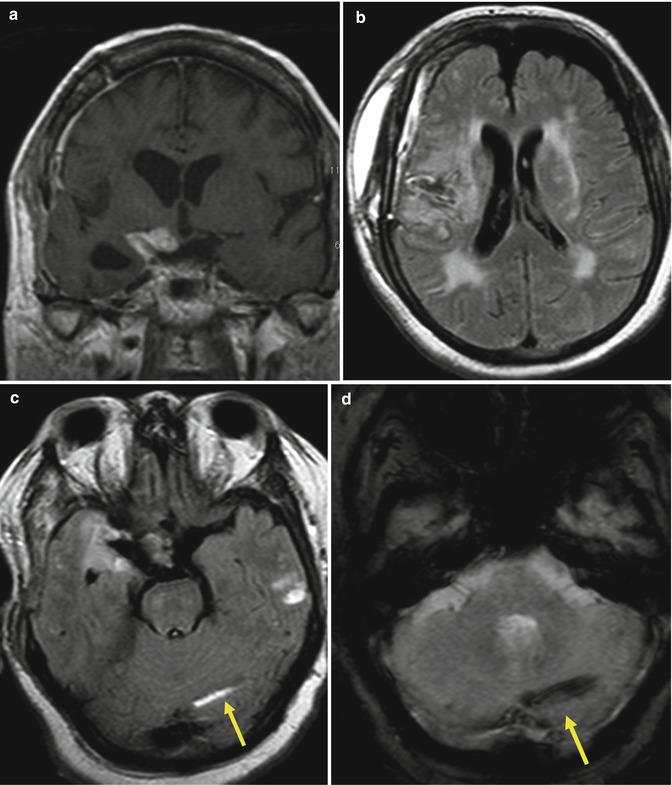
Fig. 25.2
Remote cerebellar hemorrhage in a 67-year-old woman 10 days after a pterional craniotomy for resection of a selar and right paraselar meningioma. Postcontrast T1-WI (a) and FLAIR (b) images demonstrate residual lesion and postoperative alterations at the right frontotemporal region, like scalp swelling with mixture of blood, edema, and CSF. Axial FLAIR (c) and T2 gradient echo (d) images show a left cerebellar hemisphere hemorrhage (arrow)
Craniectomy is defined as the removal of a portion of the skull, without replacement. Some complications are related to this procedure, from extracranial herniation (Fig. 25.3), subdural and subgaleal hygromas, external brain tamponade, and paradoxical herniation, a neurosurgical emergency (Fig. 25.4).
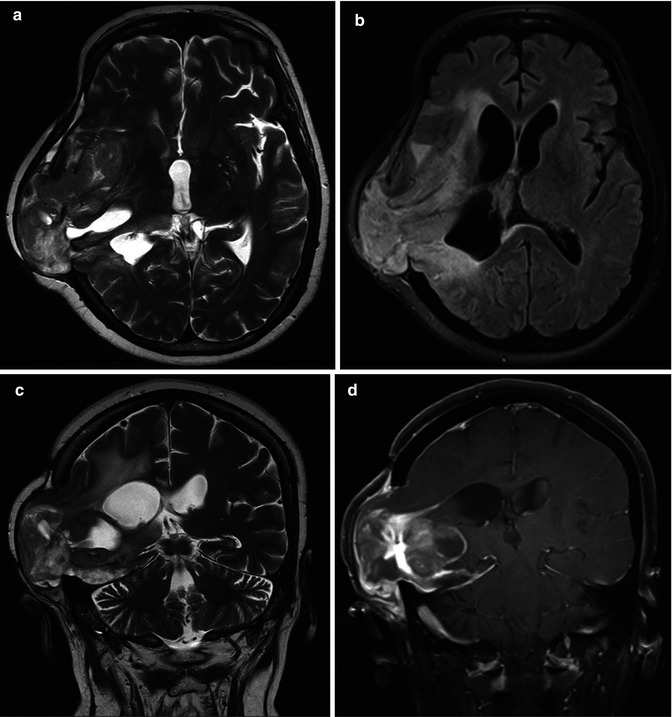
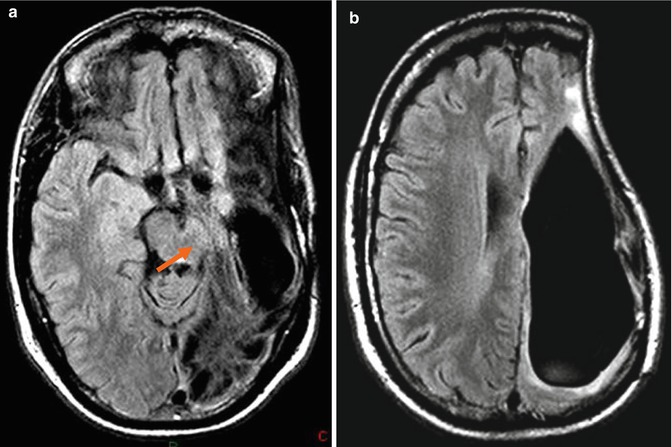
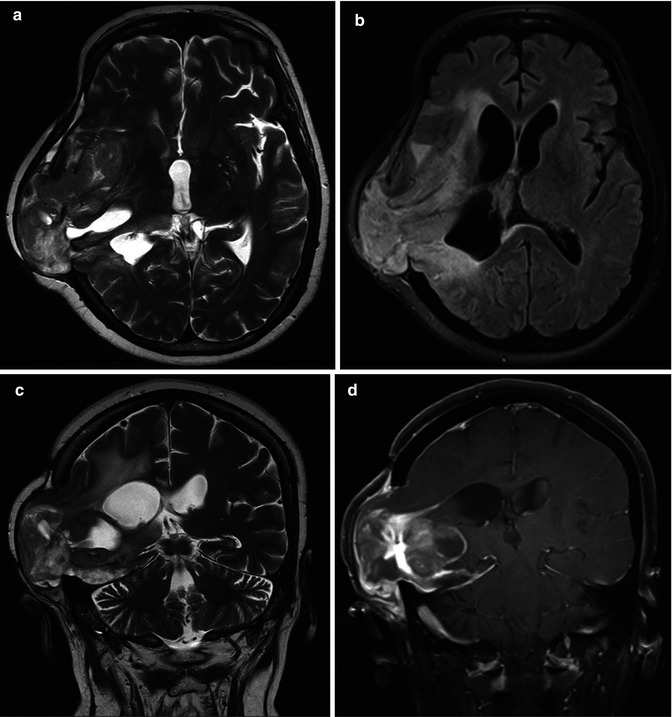
Fig. 25.3
Extracranial herniation. In a patient who underwent a partial resection of a GBM, follow-up MRI shows a brain herniating through the craniectomy. Axial T2 (a) and FLAIR (b) images, coronal T2 (c) and postcontrast coronal T1 (d) images
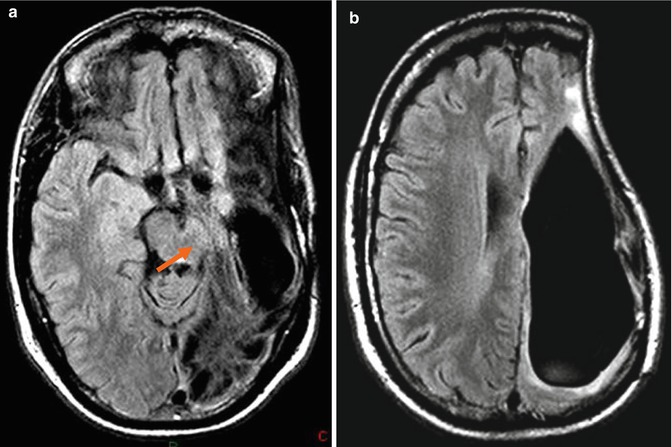
Fig. 25.4
FLAIR images (a, b) demonstrate a sunken skin flap after craniectomy for treatment of an infected bone flap, with the meningogaleal complex resting on the deformed underlying brain, as a complication of resection of an anaplastic astrocytoma. A large area of encephalomalacia is also demonstrated in the deformed underlying brain in the left cerebral hemisphere. A paradoxical herniation with a compression of the midbrain caused by a transtentorial herniation of the uncus also is seen (a, arrow)
Cranioplasty is the surgical repair of a skull defect, following craniotomy or secondary to trauma, congenital defect, or tumor, using autologous bone grafts, titanium, or acrylic. Infection is the most common complication and removal of the cranioplasty should be performed. DWI is useful for differentiating empyema from a fluid collection [1].
25.2 Early Postoperative MRI
25.2.1 Residual Contrast Enhancement
Surgical removal of a brain neoplasm is recommended with the goal of either total removal or removal of as much as possible to achieve optimal patient survival. Although changes in contrast enhancement immediately after or during treatment can mimic early tumor progression, postoperative MR imaging is the gold standard for determining the extent of tumor resection and possible existence of residual tumor.
Gadolinium-enhanced MRI proved to be extremely valuable for assessing gross residual tumor when performed at the latest 72 h after the resection of a preoperatively enhancing high-grade glioma to evaluate the extent of resection (Fig. 25.5). This timing avoided surgically induced contrast enhancement and minimized interpretative difficulties (Fig. 25.6). Therefore, contrast-enhanced MR imaging should be performed no later than 72 h after surgery to identify residual tumor in the postsurgical bed and to avoid confusion with non-tumoral inflammation [2, 3].
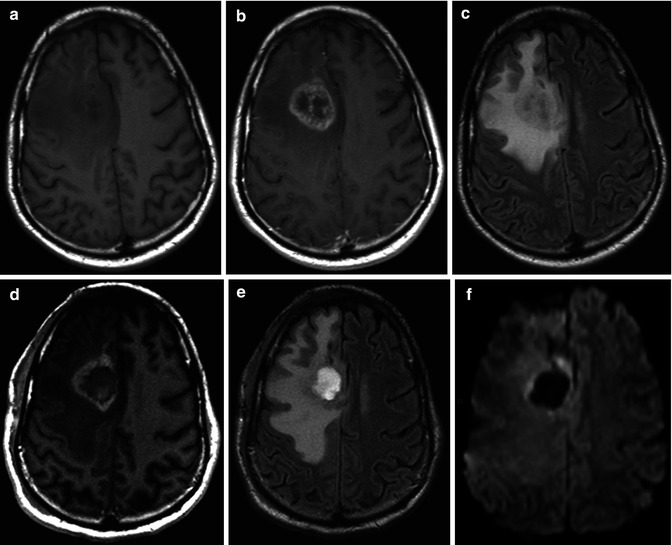
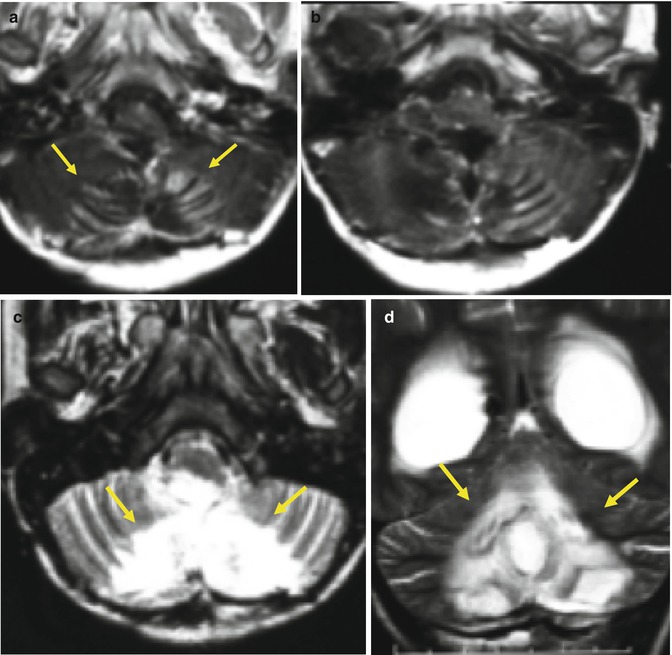
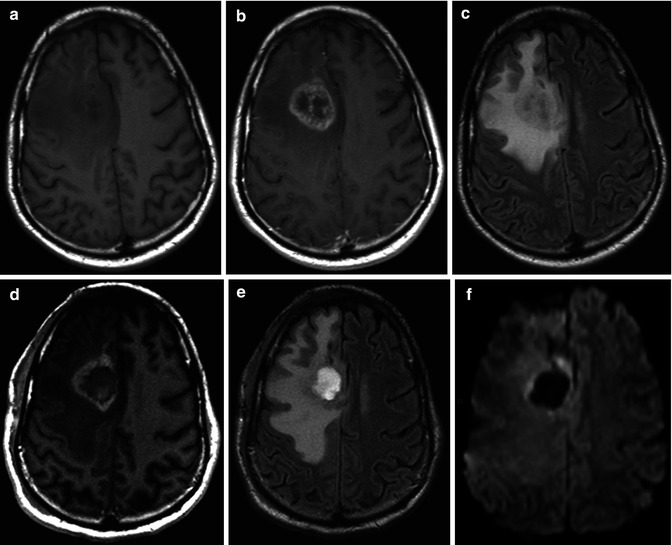
Fig. 25.5
Pre- (a) and postcontrast T1-WI (b) show a right frontal GBM in a 33-year-old man, surrounded by hyperintense area on FLAIR images (c) consisting with edema and infiltrative neoplasm. Immediate postoperative MRI performed within 36 h after surgery demonstrates residual contrast enhancement on postcontrast T1-WI (d) and FLAIR (e) images consisted with residual neoplasm. A slight area of restricted diffusion (f) is seen at the margins of the surgical cavity, which may represent cytotoxic edema related to postoperative injury
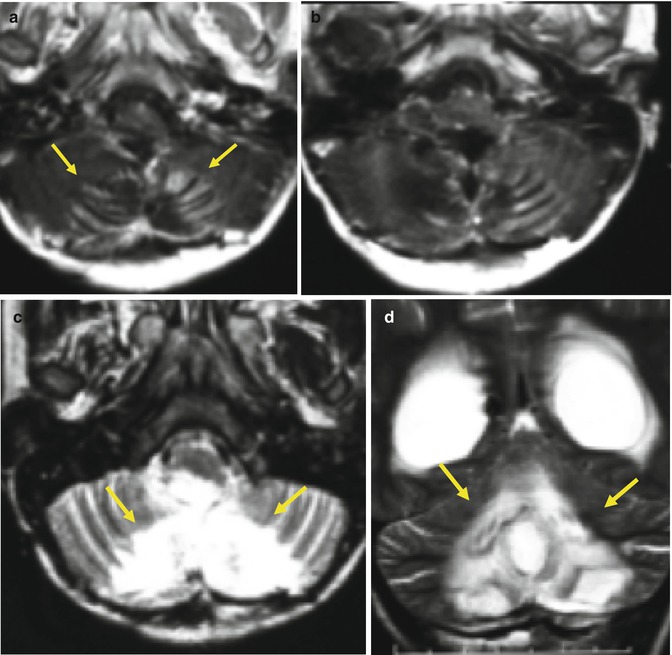
Fig. 25.6
In the follow-up MRI performed weeks after surgical resection of a pilocytic astrocytoma in a 9-year-old boy, well-delimitated areas of contrast enhancement were seen within the margins of the surgical bed (a, arrows), which may simulate the appearance of leptomeningeal neoplasm spread. In fact, these findings consist with an area of brain injury during surgery that evolved into contrast enhancement and therefore to encephalomalacia (c, d – arrows) and, thus, should not be misinterpreted as recurrent neoplasm. Axial postcontrast T1 images (a, b), axial (c) and coronal (d) T2 images
25.2.2 Diffusion-Weighted Imaging and Postoperative Injury
DWI can be very useful in the postoperative assessment of brain neoplasms. Immediately after tumor resection, an area of restricted diffusion can be observed at the margins of the resection bed as well as around it (Fig. 25.7) and remote from it (Fig. 25.8). This area invariably undergoes a subacute phase of contrast enhancement for up to 2–3 months after surgery, which can easily be misinterpreted as tumor recurrence (Fig. 25.6). However, these areas of enhancement invariably evolve into encephalomalacia or a gliotic cavity on long-term follow-up exams. These are typically thought of as postoperative ischemic changes, related to brain injury during tumor resection.
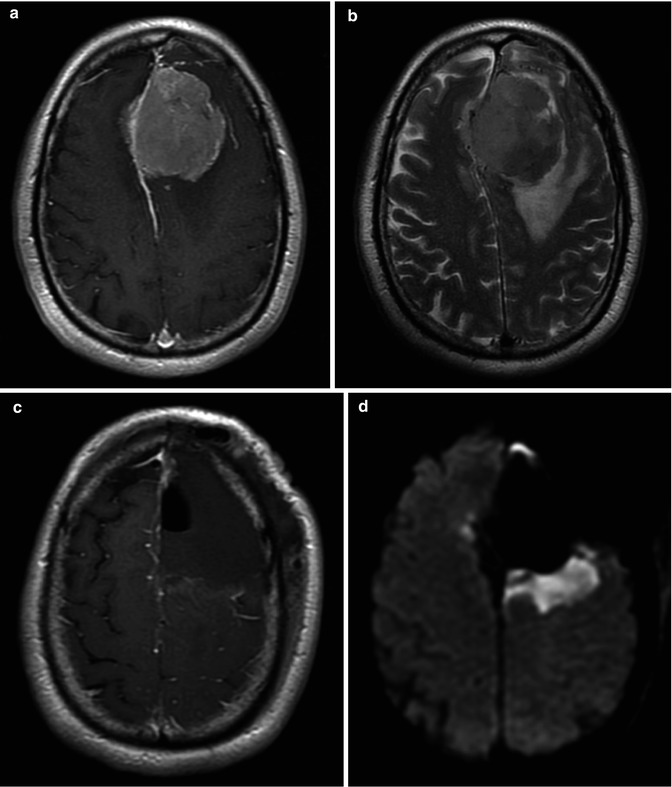
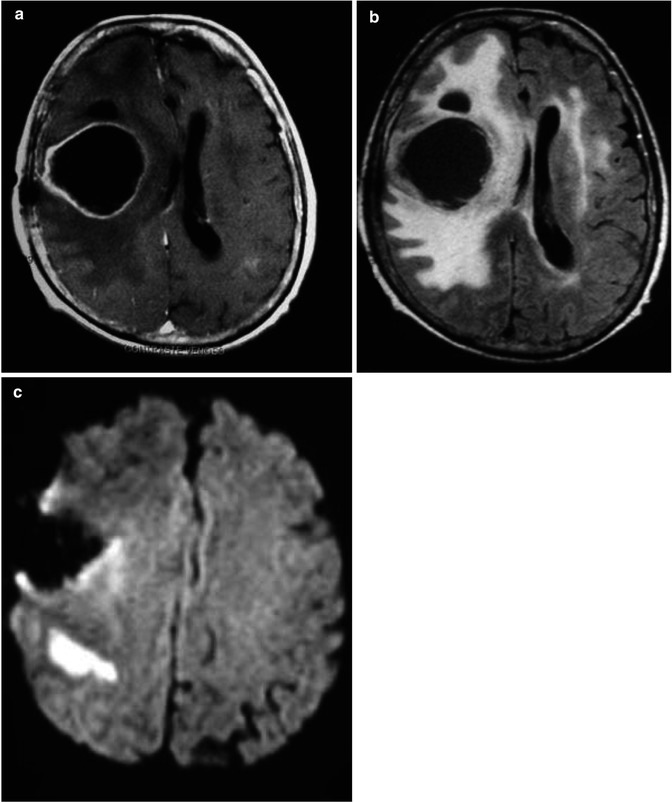
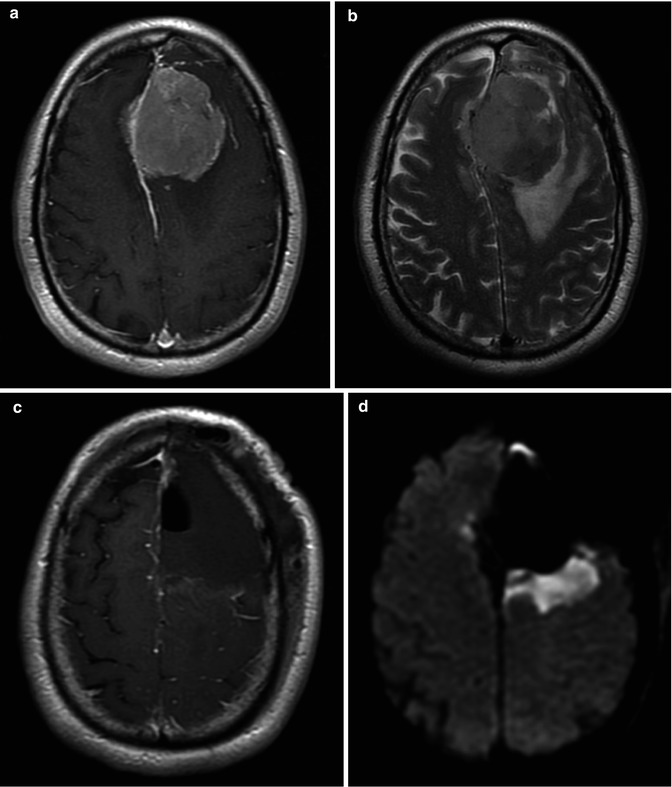
Fig. 25.7
A 69-year-old, male, with a left frontal meningioma, surrounded by vasogenic edema, which causes mass effect at the adjacent brain parenchyma (a, b). Immediate postoperative axial postcontrast T1-WI performed within 36 h postsurgery confirms gross total resection of the mass (c). Immediate postoperative DWI (d) demonstrated a wedge-shaped area of abnormal-restricted diffusion within the posterior margin of the surgical bed and a slight similar abnormality in the parasagittal right frontal lobe, consisting with acute brain injury related to surgery
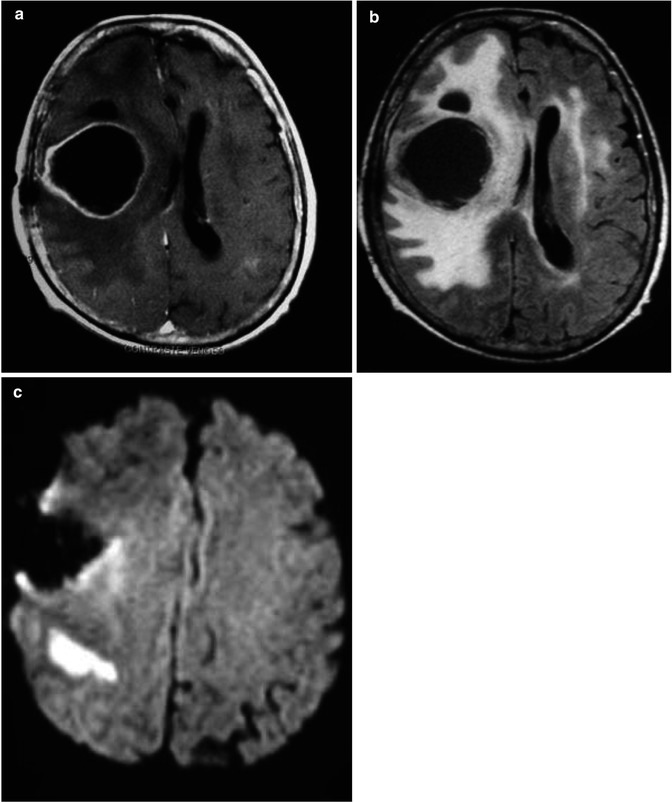
Fig. 25.8
A 51-year-old male with GBM. MRI demonstrates an expansive ring-enhancing lesion on the postcontrast T1-WI (a), with necrotic center and surrounded by hyperintense area on FLAIR images (b). The immediate postoperative follow-up DWI (c) demonstrates area of restricted diffusion posteriorly to surgery bed, consisting with acute brain injury related to surgery
Thus, DWI should be performed during the immediate postoperative MRI exam to recognize areas of brain injury. As these areas in the follow-up exams may present with contrast enhancement, they can be confused with recurrent tumor and interpreted erroneously as treatment failure. Hence, a new area of contrast enhancement observed on postoperative MRI should be interpreted in the context of the immediately postoperative DWI [3, 4].
25.3 Tumor Progression on Conventional MRI
25.3.1 FLAIR Signal intensity Within the Resection Cavity
The current standard for first-line treatment of gliomas is complete resection of the tumor. After the surgical approach, a resection cavity filled with normal cerebrospinal fluid (CSF) is seen. Some authors have observed that an alteration in the signal intensity of the glioma resection cavity in FLAIR images might be able to indicate subsequent or coincident tumor progression (Fig. 25.9). This observation could be explained by the fact that slight tumor growth at the margins of the resection cavity is not yet detectable by standard imaging analysis. There is a higher protein concentration due to disturbance in CSF exchange, hemorrhage, as well as some neoplasm cells and other cell debris within the cavity. Hence, increased signal intensity within the resection cavity can help predict subsequent tumor growth [5].
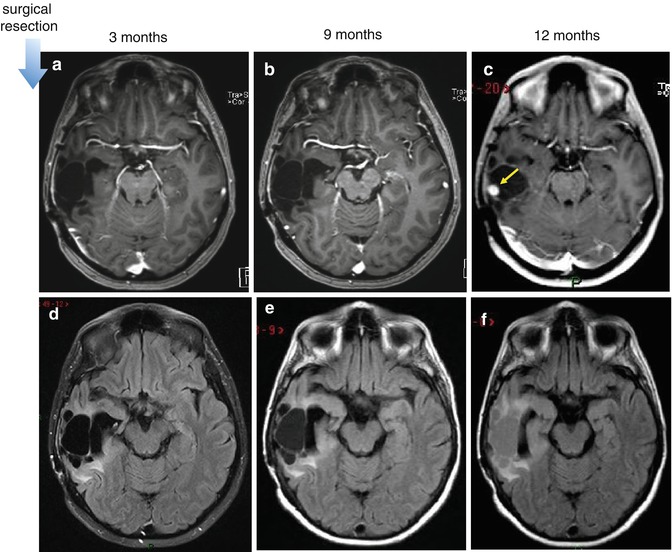
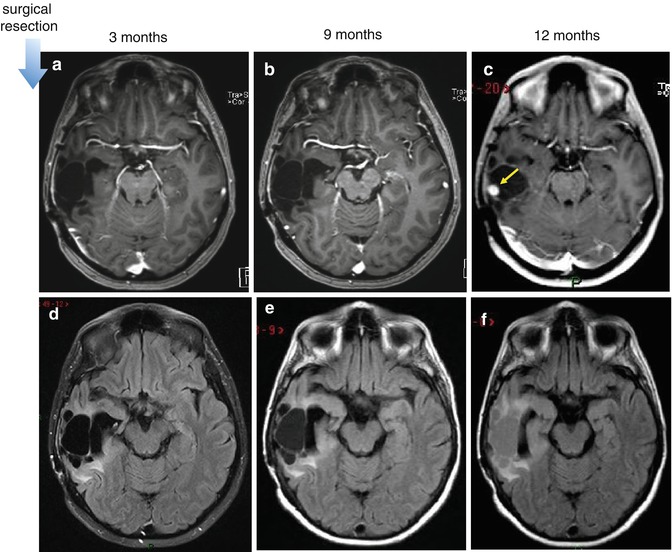
Fig. 25.9
Progression neoplasm. Increase in signal intensity within the surgical cavity on FLAIR images, before appearance of contrast-enhancing lesion and progression of tumor size. First row is postcontrast T1-WI (a–c) and second row is FLAIR images (d–f). Postoperative follow-up MR images in a patient with resect glioma in the right temporal lobe. The increase in signal intensity within the surgical cavity on FLAIR images (e) is visible in the exam performed 9 months after surgery, whereas progressive contrast-enhanced recurrent lesion is only seen 3 months later (c, arrow)
25.3.2 Tumor Size: Longest Tumor Diameter
Several areas of MR imaging have advanced, incorporating new knowledge regarding modern contrast media, functional techniques, and advanced morphologic approaches. However, in daily practice, routine MRI using conventional sequences to determine tumor size is still an important and simple surrogate marker of tumor response to treatment [5] (Fig. 25.10).
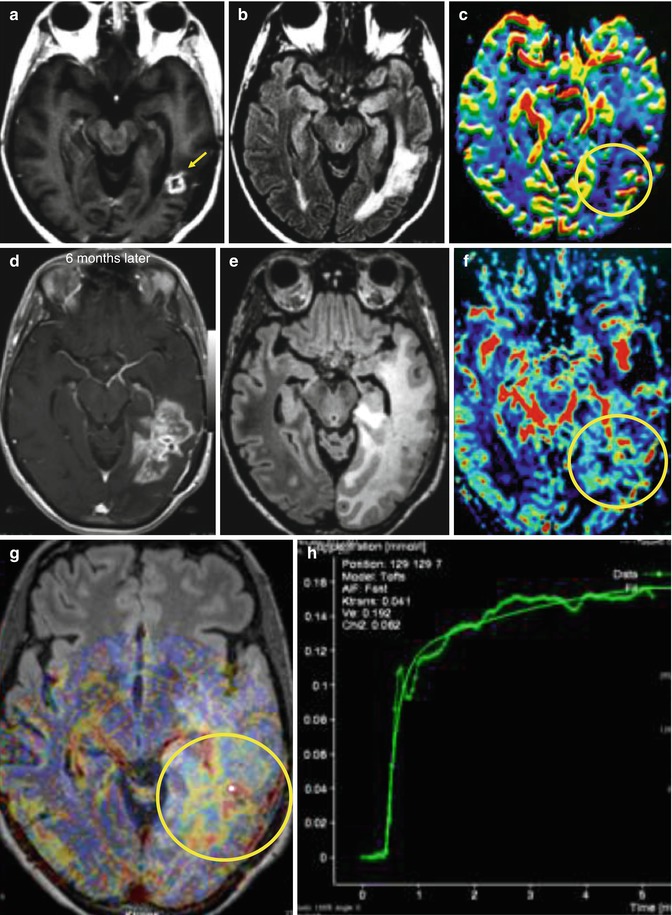
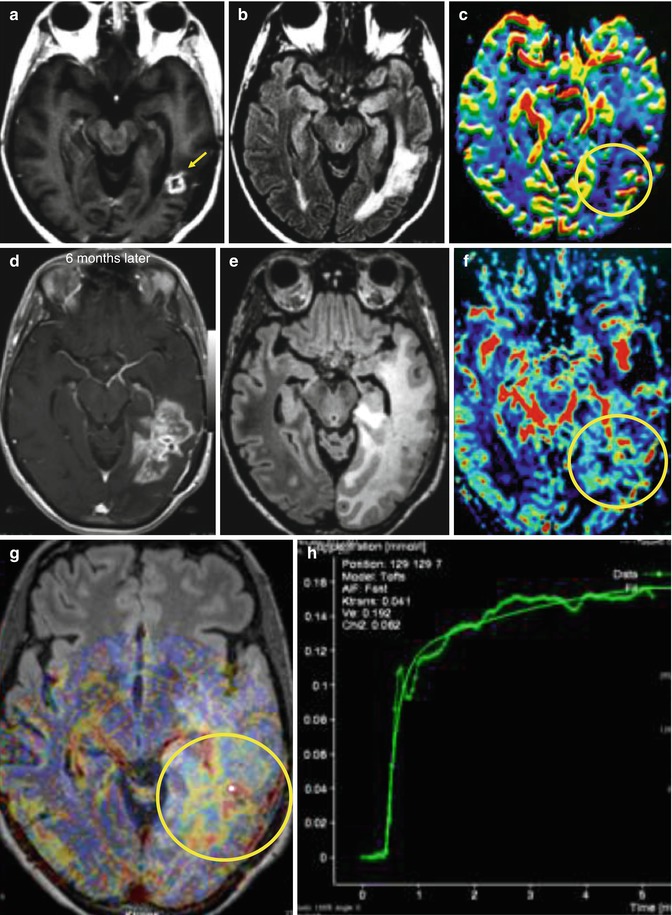
Fig. 25.10
MR exam after resection of GBM, followed by radiation therapy, shows a residual-enhancing lesion in the postcontrast T1-WI (a, arrow), surrounded by hyperintense area on FLAIR images (b), without presenting hyperperfusion on rCBV map (c, circle). Six months after treatment, patient got worse clinically, complaining of headache and seizures. Recurrent tumor was diagnosed in the follow-up MR exam, as there was an enlargement of the enhancing lesion on postcontrast T1-WI (d) and the surrounding abnormal hyperintensity on FLAIR images (e), with hyperperfusion on rCBV maps (f, circle) and high permeability (g, h, circle)
25.4 Treatment-Induced Changes
25.4.1 Introduction
Accurate diagnosis of treatment-induced changes is critical in order to exclude other potentially treatable disorders, to prevent unnecessary diagnostic procedures and inappropriate antineoplastic therapy, and to allow meaningful prognostication. Many of the deleterious effects of radiation and chemotherapy are well known. These include early effects, such as vasogenic edema, which occur within a few weeks of the treatment and delayed effects that can occur months to years later. The latter effects include cerebral atrophy, necrosis, demyelination, gliosis, and neoplasm [6].
25.4.2 Current Treatment of High-Grade Gliomas
The current standard of care of high-grade gliomas is surgical resection followed by radiotherapy (RT) and concomitant and adjuvant temozolomide (TMZ) chemotherapy. It represents a milestone in the high grade glioma treatment, because this approach has been shown to prolong the overall survival of these patients [7].
The goal of surgery is to establish the diagnosis, perform maximum tumor resection, and decrease the size of the mass. Following surgery, patients are treated with radiation therapy, often with concurrent chemotherapy using temozolomide, an oral alkylating agent, continued for up to 6 months after surgical intervention [7]. Fractionated radiotherapy has been shown to extend survival in multiple randomized trials, and the concomitant use of temozolomide has proven superior to radiation alone [7].
Whereas current standard treatment tends to be fairly consistent, treatment at the time of recurrence needs to be individualized, based on the patient’s disease status, performance status, and the tumor anatomy. Treatment options for tumor recurrences include repeat surgery with or without local chemotherapy, standard chemotherapy regimens, brachytherapy, or a combination of these.
25.4.3 Radiation Necrosis vs Tumor Recurrence: A Diagnostic Dilemma
The differential diagnosis between tumor recurrence and treatment effects has become an important and difficult diagnostic dilemma in clinical practice. During or following treatment, nonenhancing gliomas may initially develop enhancement. The development of new enhancement areas on the tumor resection margins or distant from it could potentially be attributable to either recurrence or radiation necrosis.
Conventional MR imaging findings are considered insufficient for reliably distinguishing radiation necrosis from tumor recurrence, which has confounded differential diagnostic evaluation. Radiation necrosis can present similar conventional MR imaging characteristics of gliomas, including contrast enhancement, mass effect, and vasogenic edema [8]. However, some findings can favor recurrent tumor, like involvement of the corpus callosum crossing of the midline associated with subependymal spread and/or multiple lesions.
Use of advanced imaging techniques, such as perfusion- [9] and diffusion-weighted MR imaging [10, 11] and MR spectroscopy [12], has suggested increased sensitivity and accuracy compared with conventional MR imaging. Nonetheless, there is no advanced MR technique that could precisely make such a differentiation. Moreover, conventional MR serial imaging can be helpful in this differentiation.
25.4.4 Radiotherapy
25.4.4.1 Vascular Malformations (Capillary Telangiectasia and Cavernoma)
Cerebral delayed radiation injury can be manifested as vasculopathy triggered by injury to the cerebral microcirculation. Some reports have attributed the formation of cavernomas and capillary telangiectasia to radiotherapy. Radiation injury leads to hyalinization and fibrinoid necrosis of vascular walls, resulting in occlusion and infarction as well as vascular proliferative lesions such as capillary telangiectasia and cavernoma. However, the exact mechanisms of this induction are complex and poorly understood [13, 14].
In about 20 % of children who undergo cranial irradiation, capillary telangiectasia occurs within 3–9 months after therapy, and in most cases they are asymptomatic [15, 16]. As the pediatric brain is more sensitive to radiation than the adult brain, telangiectasia is thought to affect more children. Cavernoma has a longer latency period, ranging from 1 to 26 years to develop [13], and is associated with increased risk of hemorrhage [17] (Fig. 25.11).
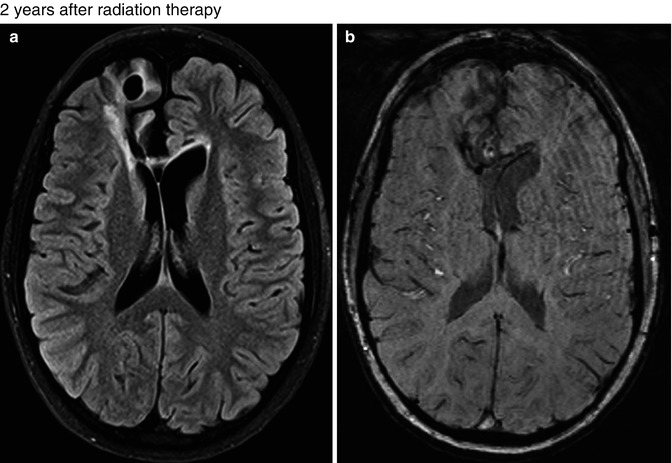
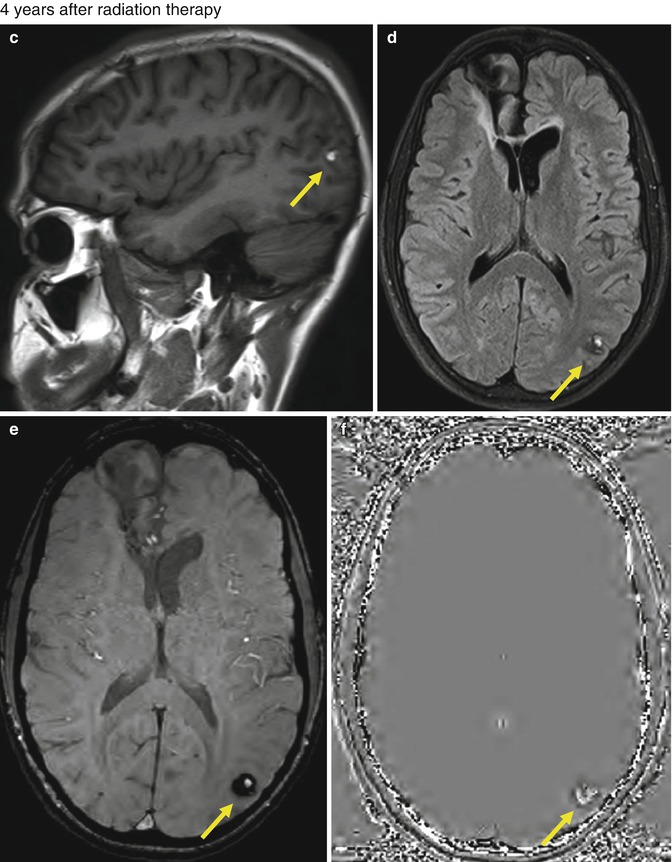
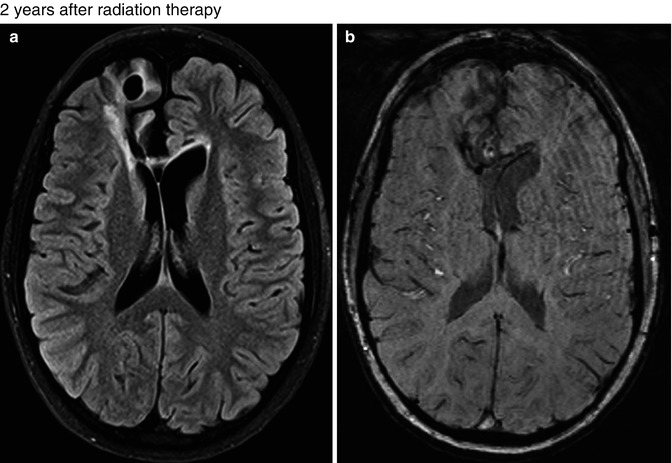
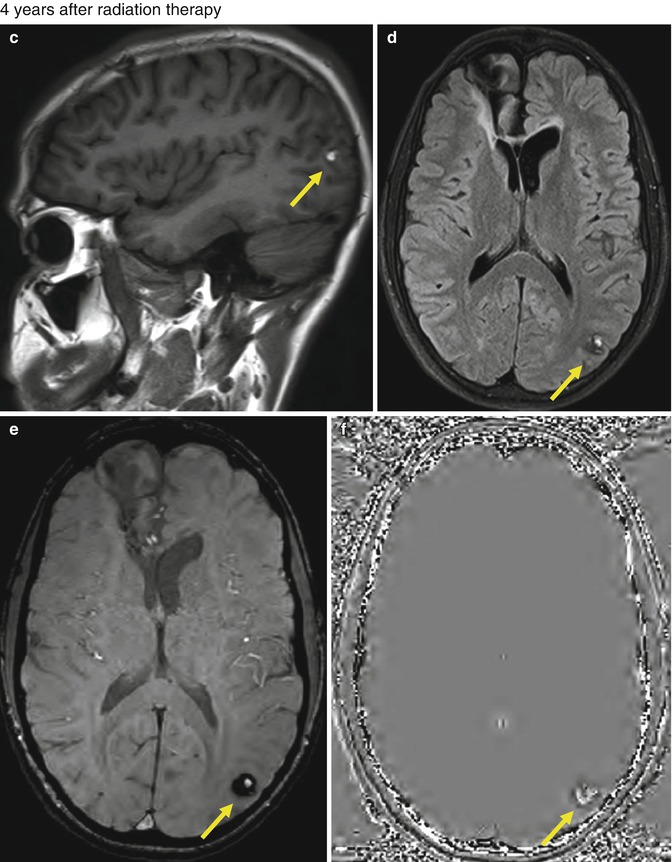
Fig. 25.11
A 15-year-old boy with germinoma was treated with surgery followed by radiation therapy and chemotherapy. FLAIR (a) and SWI (b) images obtained 2 years after treatment show postsurgery changes at the right frontal lobe, and no lesion is seen in the left occipital lobe. Axial T1-WI (c), FLAIR (d), SWI (e), and SWI phase (f) images obtained 4 years after the radiation therapy showing a round lesion of mixed signal intensity with peripheral hypointense rim in the right occipital lobe, suggestive of cavernous angioma (arrows). No other lesion was seen
It is not well known whether these vascular abnormalities are associated with higher radiation doses or not. Prior reports are ambiguous and a true association is not clear. Capillary telangiectasia has not been significantly associated with a higher dose, although there has been a trend in this direction [15]. A correlation between the radiation dose and a shorter latency to development of cavernomas has been demonstrated [18].
25.4.4.2 Radiation-Induced Meningioma
Radiation-induced meningioma is the most common form of radiation-induced neoplasm [19]. Diagnostic criteria for a radiation-induced meningioma include a tumor arising in the irradiated field; histological features different from those of a previous neoplasm; a sufficient latency period, usually more than 5 years; no family history of phakomatosis; and absence of tumor prior to radiation therapy.
Radiation-induced meningioma tends to occur in younger individuals, after variable latency periods, with a higher incidence of multiplicity, higher recurrence rate after treatment, and an aggressive behavior [20]. Even though those tumors are considered benign, they more frequently have a potential for rapid growth and aggressiveness.
The age of patients and the latency periods in radiation-induced meningiomas are thought to be dose related, and younger individuals at the time of irradiation have shorter intervals to meningioma formation [20–22]. Nevertheless, the true incidence of radiation-induced meningiomas is presently unknown.
Treatment of choice for most cases is surgical removal with a wide bone and dural margin resection to avoid recurrence. However, in some cases, complete resection may not be possible due to multiplicity, involvement of osseous structures and vessels, as well as its aggressiveness [22].
25.4.4.3 Radiation-Induced High-Grade Glioma
Development of a radiation-induced neoplasm is a relatively rare delayed complication of radiation therapy. Although sarcomas and meningiomas represent the most frequent tumors that appear, high-grade gliomas may also develop in the previously irradiated field [23].
No threshold radiation dose has been identified for malignant transformation. The risk of developing a secondary malignancy within the original field of irradiation increases with radiation doses [24]. Thus, a majority of the secondary malignancies appeared to fall within the high-dose region of radiation.
No histopathologic differences between radio-induced gliomas and so-called “primary” gliomas were identified. In most cases, patients affected are younger and usually have a malignant histotype, which may be situated in cerebral compartments rarely affected by the primary glioma, such as the suprasellar region and the cerebellar fossa (Fig. 25.12).
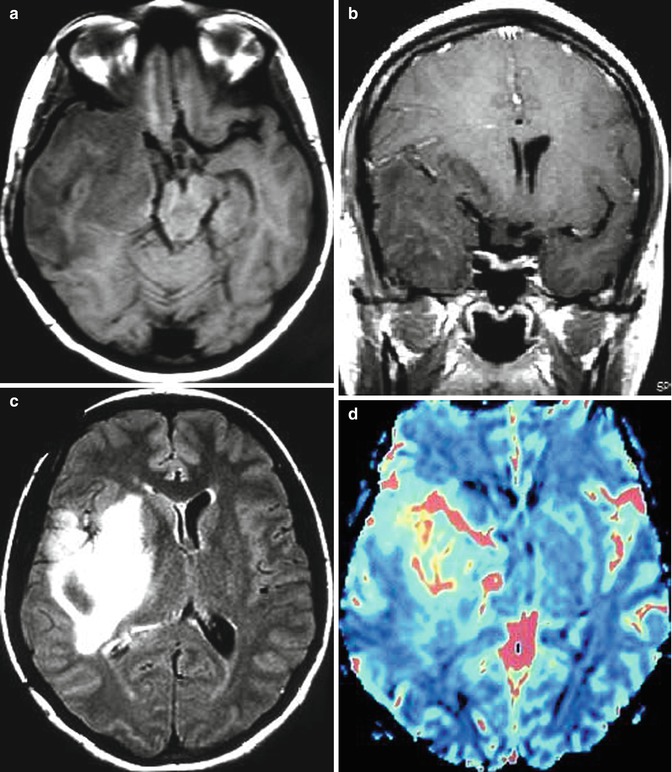
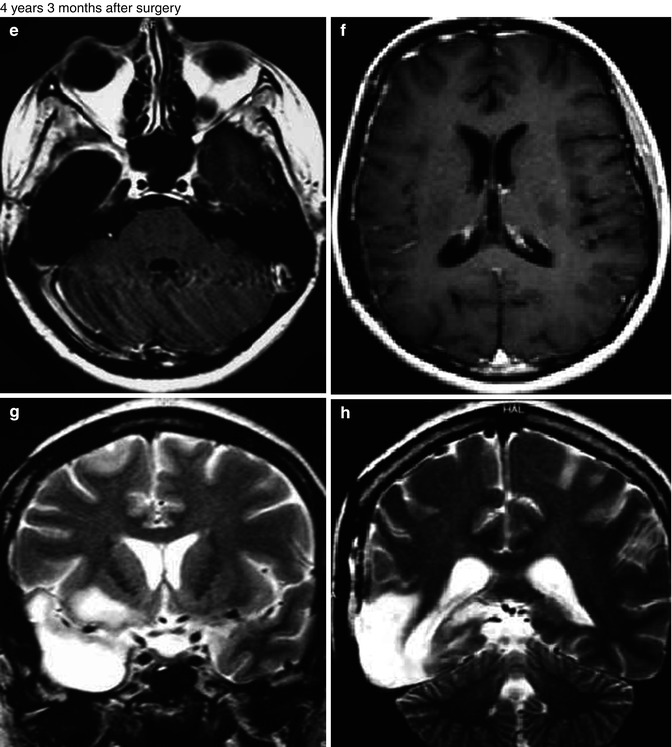
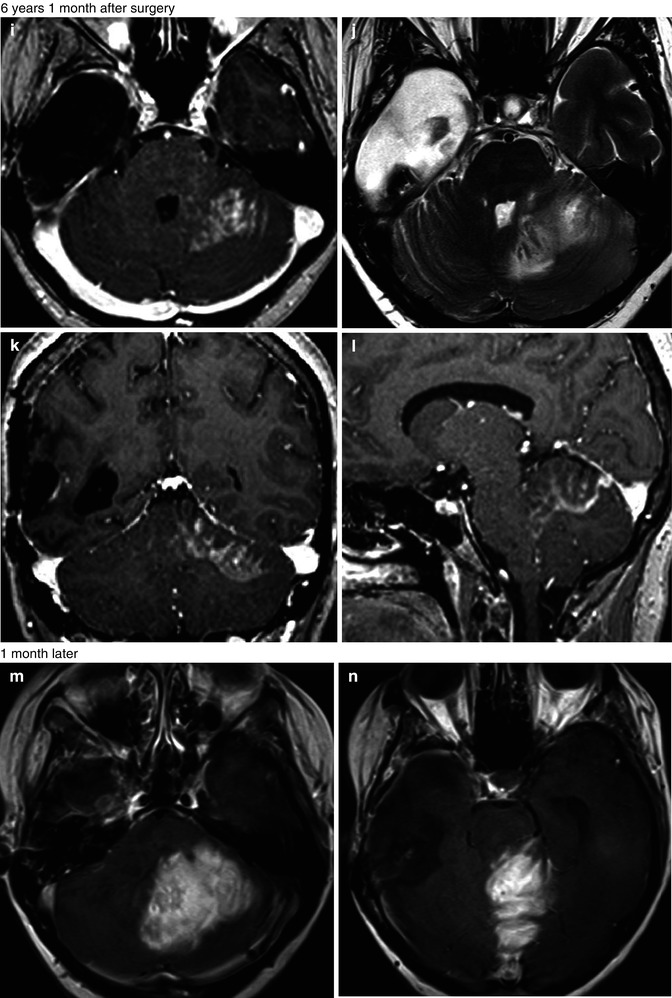
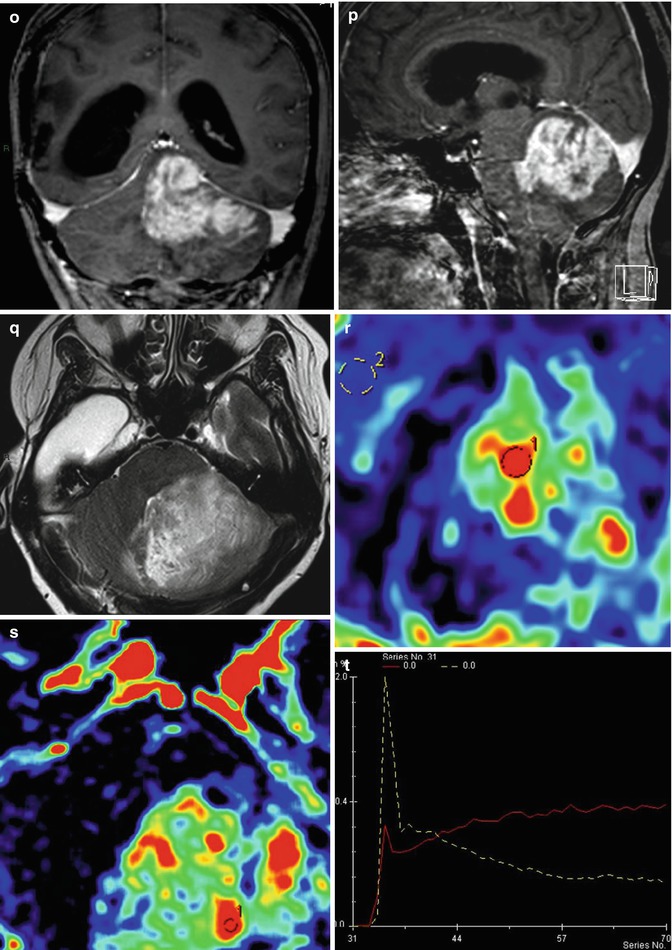
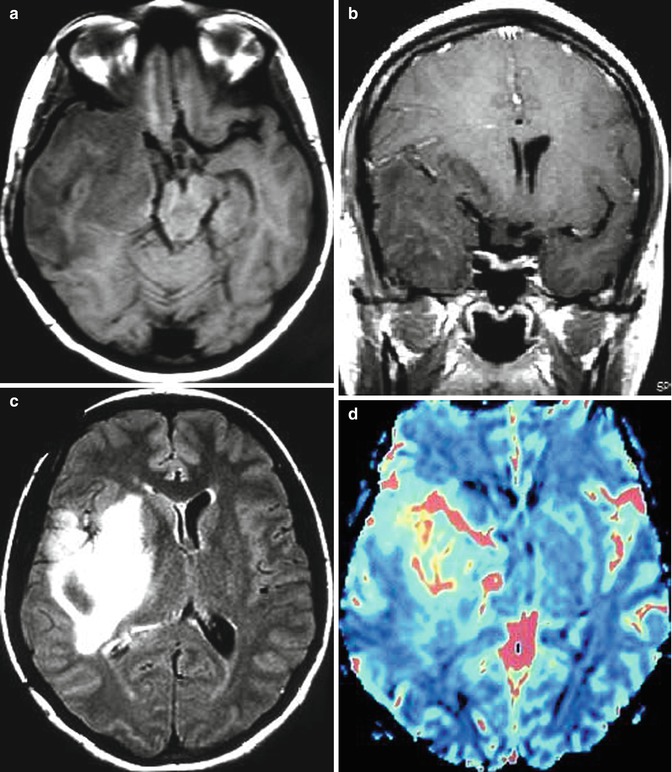
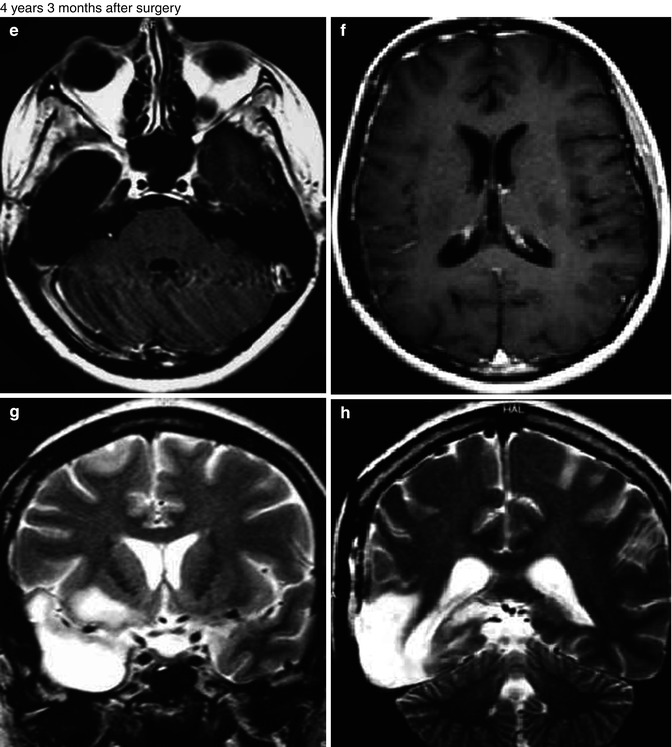
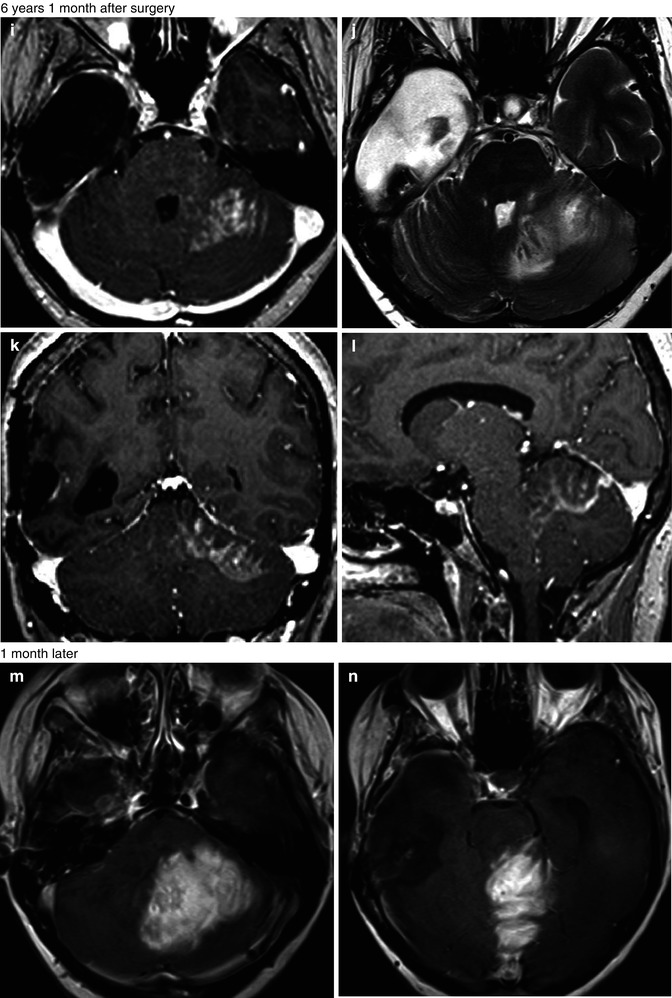
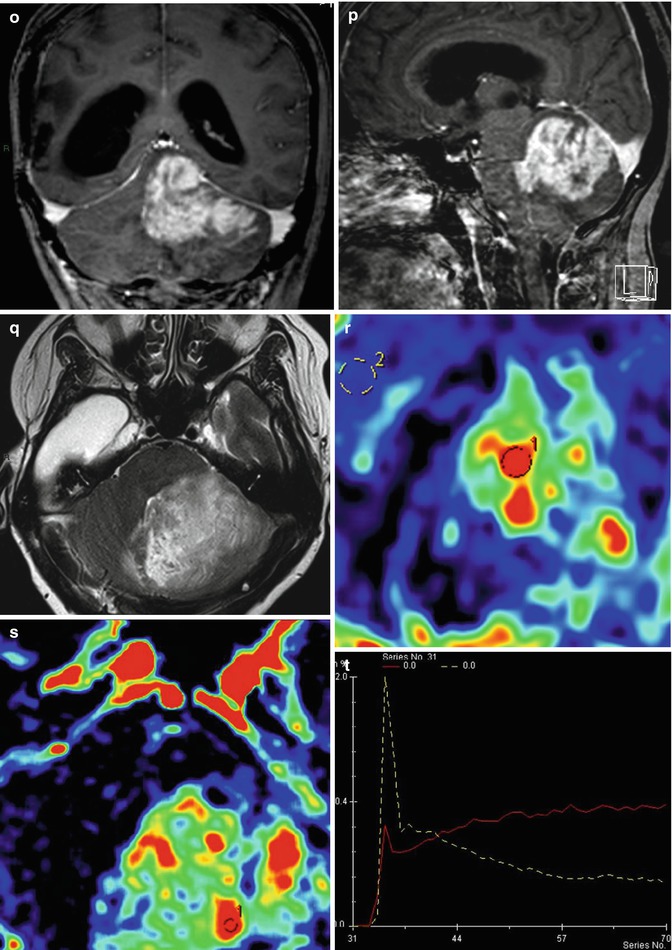
Fig. 25.12
Radiation-induced high-grade tumor. 37-year-old, woman, with an anaplastic astrocytoma, was submitted to surgery followed by radiation and chemotherapy. MRI showed an expansive lesion, with no contrast enhancement (a–c), but presenting hyperperfusion on rCBV map (d). Four years and 3 months later, the patient was asymptomatic and no lesion was observed in the follow-up MRI (e–h). Almost 6 years after the radiation therapy, the patient got worse clinically, presenting mental confusion, seizures, gait disturbance, headache, and vomiting. The MRI (i–l) demonstrated a new expansive and contrast-enhancing lesion at the left cerebellum hemisphere, in the field of the radiotherapy. In the MRI obtained 1 month later (m–t), the contrast-enhancing lesion enlarged and presents hyperperfusion on rCBV map (r) and a high permeability (s, t)
25.4.4.4 Radiation-Induced Leukoencephalopathy
Radiation-induced leukoencephalopathy is a long-term complication that can occur months or years after radiotherapy. Its estimated incidence varies from 2 to 50 % and generally occurs after whole-brain irradiation [25, 26]. Symptoms include progressive mental slowing and cognitive decline and personality change, leading to frontal–subcortical dementia. It also may be accompanied by gait difficulties, dopa-resistant parkinsonian syndrome, ataxia, headache, and urinary incontinence [27]. However, despite the sometimes extensive white matter lesions and the wide range of associated symptoms, in some particular cases, patients may be asymptomatic.
Initially MR imaging shows progressive subcortical atrophy and enlarged ventricles, followed by periventricular white matter changes that evolve to confluent white matter abnormalities (Fig. 25.13). The degree of abnormality on MR imaging has been correlated with cognitive deficits [25].
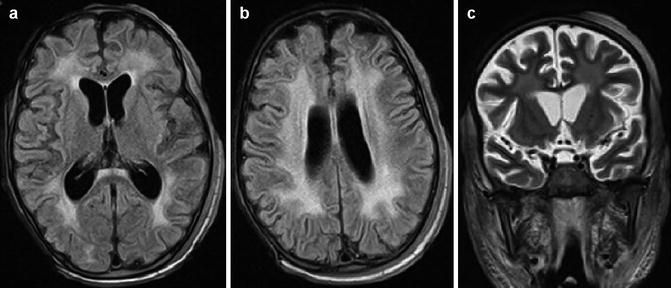
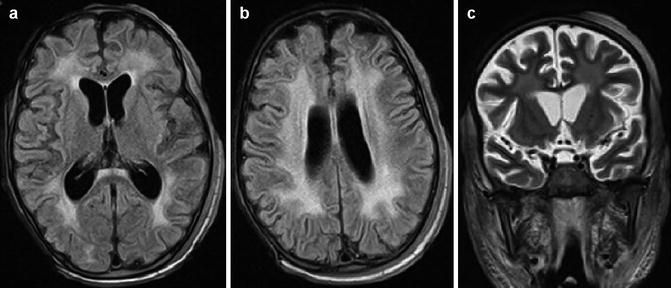
Fig. 25.13
Radiation-induced leukoencephalopathy in a child with acute lymphoblastic leukemia submitted to whole-brain radiotherapy. FLAIR images (a, b) and T2-WI (c) show diffuse white matter injury associated with brain atrophy. Also note radiation-induced necrosis at the scalp of the right hemisphere well as diffuse swelling of adjacent soft tissues
The risk of radiation-induced leukoencephalopathy appears to be related to the total dose of radiation, larger dose per fraction, concomitant use of chemotherapy, age at the time of radiotherapy, and coexisting white matter disease, such as multiple sclerosis or leukoaraiosis [26]. Depending on the severity, the lesions may partially resolve, stabilize, or progress, leading to widespread, potentially fatal, brain destruction characterized by coagulation necrosis. As no specific therapies are known to reverse its progressive course, treatment is palliative.
25.4.4.5 Radiation Necrosis
Radiation necrosis as a complication of brain tumor treatment is not uncommon, with an incidence ranging from 5 to 24 %. Usually seen from 2 to 32 months after therapy, 85 % of cases occur within 2 years [28].
Histologic features of radiation injury include reactive white matter edema, demyelination, fibrinoid changes in the blood vessels, coagulative necrosis, cysts with central liquefaction, and gliosis in the walls. Ischemic injury can lead to blood–brain barrier disruption and necrosis. The normal-appearing brain tissue that surrounds the tumor margin can present with transient enhancement, secondary to blood–brain barrier breakdown, and can be mistaken for tumor enlargement. The enhancing portion of the radiation necrosis can demonstrate a temporary increase in size, which tends to occur 3–12 months after radiation therapy and diminish within 5–7 months after onset.
With conventional MR imaging, enlargement of an enhancing lesion and worsening of vasogenic edema following radiation therapy may be due to radiation necrosis or tumor progression. Temporal correlation is an important feature for assessing a brain tumor lesion treated with radiation, as a latent period of less than 2 years suggests radiation induction. Conventional MR imaging can also demonstrate some aspects suggestive of radiation necrosis. Lesions greater than 2 cm have a tendency to show a central necrotic core, rim enhancement, and fingerlike projections, with a “soap bubble” appearance, whereas small lesions tend to form solid homogeneously enhanced nodules [28] (Fig. 25.14). Besides these observations, evaluation with more advanced imaging techniques may be desirable.
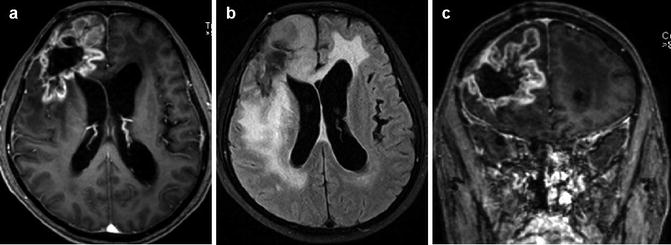
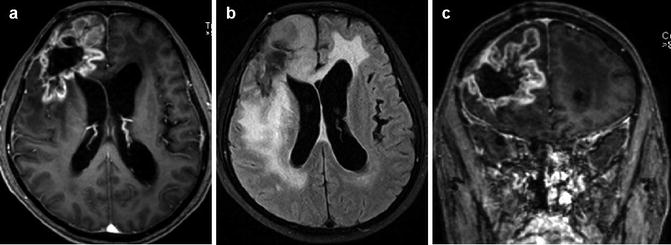
Fig. 25.14
Radiation necrosis after treatment of GBM. Postcontrast T1-WI (a, c) and FLAIR (b) images demonstrate a right frontal expansive lesion, with a peripheral and irregular contrast enhancement, with appearance of “soap bubble,” and a central necrotic area
As residual/recurrence tumor and necrosis can coexist at same time, complete differentiation is frequently impossible. Serial MR imaging and stereotactic biopsy have been used traditionally as primary methods of diagnosing radiation necrosis. In the evaluation of radiation necrosis, serial MR imaging follow-up can be useful to confirm resolution or stability. Some typical radiation-induced changes have been described, such as progressive confluent hyperintensity in the white matter, periventricular enhancing foci distant from the resection cavity but within the radiation port, and distant enhancement not within the expected pathways of tumor spread within the radiation port.
25.4.5 Chemotherapy
25.4.5.1 White Matter Injury
Chemotherapy-induced CNS toxicity has been observed with increasing frequency, maybe due to the growing use of aggressive chemotherapeutic agents, the dose administered, and the use of different routes of administration. The white matter is by far the most common site involved, especially myelin. If methotrexate is administered intravenously, less than 10 % of patients develop leukoencephalopathy. However, this complication is seen in up to 40 % of patients receiving intrathecal methotrexate [29, 30].
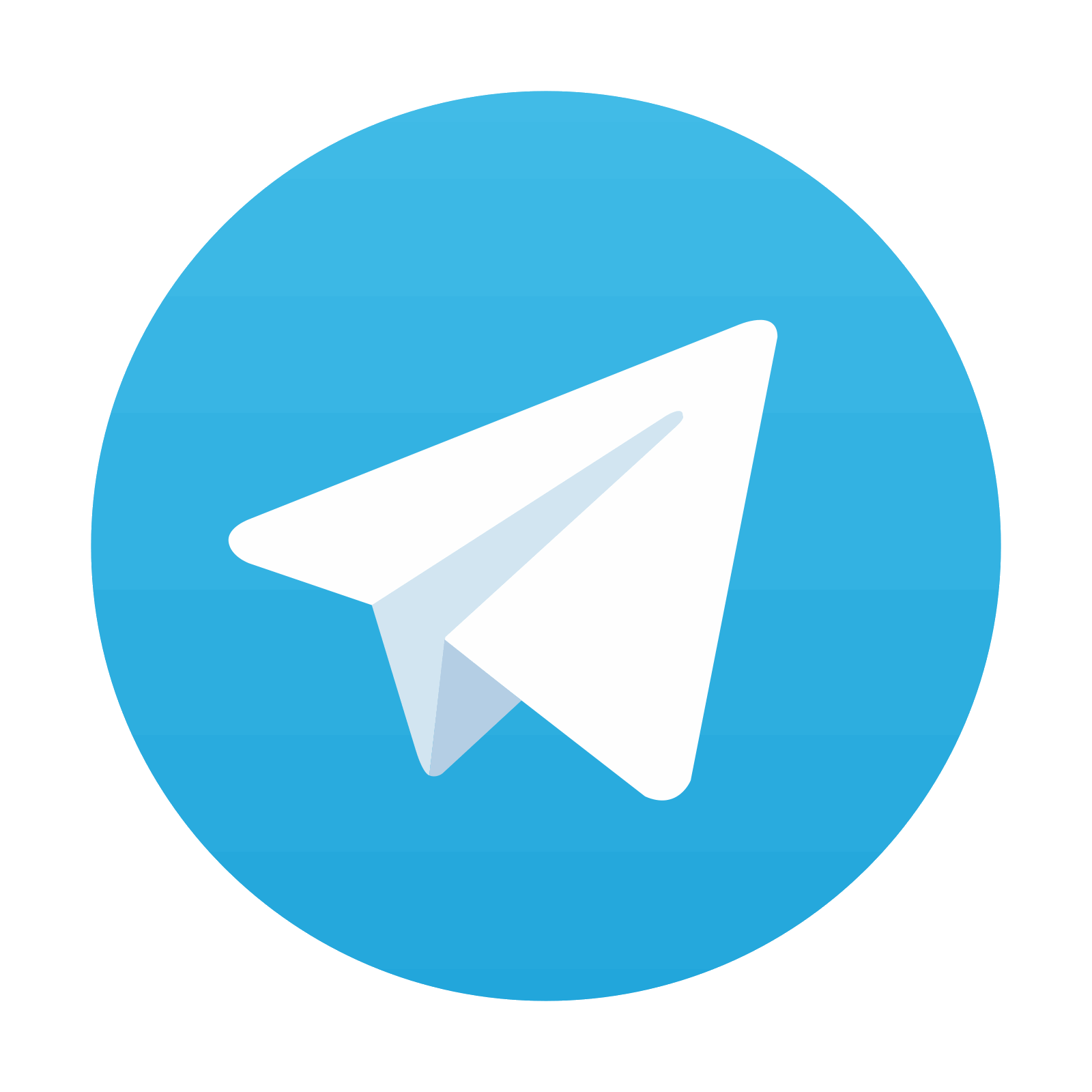
Stay updated, free articles. Join our Telegram channel
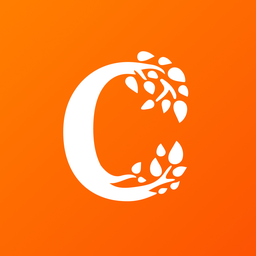
Full access? Get Clinical Tree
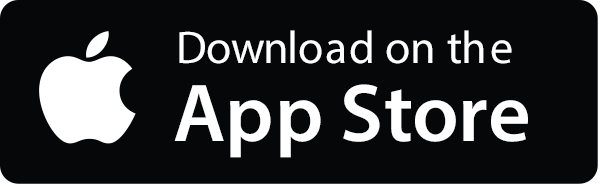
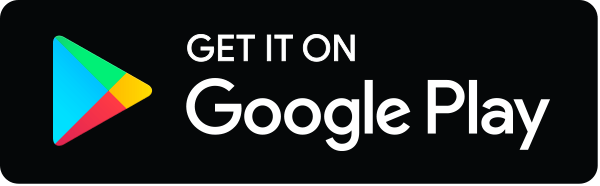