MRI has historically been underused to evaluate the lungs and airways in children because of challenging technical factors leading to suboptimal image quality. Advances over the past several years have made MR imaging a feasible option to evaluate various disorders of the chest in pediatric patients. This review article discusses up-to-date techniques and protocols for MR imaging of the lungs and airways in children. MR imaging findings in commonly encountered disorders of the lungs and airways are also reviewed.
Key points
- •
MR imaging of the lung and airways in children is feasible for many clinical applications.
- •
MR imaging may be used as an alternative to CT to evaluate several congenital lung and large airway disorders and infectious lung disorders in children.
- •
Effective MR imaging of the lungs and airways can be performed using MR imaging scanners and sequences already available in most tertiary medical centers.
Video content accompanies this article at http://www.mri.theclinics.com .
Introduction
Cross-sectional imaging is frequently indicated to evaluate various disorders of the lung and airway in the pediatric population. Although MR imaging is often the preferred modality when imaging other organ systems, computed tomography (CT) has historically been favored when imaging the lung and airways due to technical challenges inherent in obtaining high-quality MR images of the chest. However, recent advances in MR imaging technology have strived to overcome these challenges and establish MR imaging as a potential alternative to CT for certain clinical indications in infants and children. This article presents up-to-date MR imaging protocols for imaging disorders of the lung and airway in the pediatric population. Scenarios when MR imaging might be considered are described and imaging findings are illustrated.
Imaging techniques
Patient Preparation
Patient preparation for MR imaging is highly dependent on the patient’s age. In infants, a “feed-and-wrap” technique may be used, in which the child is fed and wrapped in a blanket or vacuum mattress, keeping him/her still for the examination. For patients aged 18 months to 5 years, sedation is usually required to avoid motion artifact. Sedation is usually achieved using one of the following medications: fentanyl, midazolam, pentobarbital, propofol, chlorpromazine, or sevoflurane. Children as young as 5 years of age may not require sedation if properly coached, although this is age variable and dependent on each child’s abilities. For lung and airway MR imaging, training can be combined with the use of an MR imaging-compatible spirometer. MR imaging success rates increase when allowing a parent to stay with the child and using distraction methods such as MR imaging-compatible movie goggles.
MR Imaging of the Lungs
The use of MR imaging to evaluate disorders of the lungs has received increased attention during the last decade. Technological advances have made it possible to perform chest MR imaging with diagnostic image quality in a reasonable scan time. Basic MR imaging protocols including a combination of spin echo and gradient echo sequences can provide image quality similar to that of CT. Moreover, new sequences such as ultrashort echo time (UTE) or zero echo time (ZTE) seem to have filled the gap with CT regarding image quality. These sequences can be easily combined with respiratory gating techniques, using a pneumobelt or navigator echo, that facilitate MR imaging scanning in non-cooperative children. Further reduction of motion artifacts with these free-breathing techniques is achieved using helicoidal (PROPELLER@GE) or radial (STARVIBE@SIEMENS) k-space acquisition schemes. Although CT is often regarded as the gold standard in cross-sectional thoracic imaging, MR imaging has the potential to provide functional information about ventilation, inflammation, perfusion, and structure in a single examination, which has been summarized under the acronym of ventilation, inflammation, perfusion, and structural (VIPS)-MR imaging protocol. A basic and advanced ventilation, inflammation, perfusion, and structural-MR imaging protocol is presented in Table 1 .
Sequence | Manufacturer Acronyms | Typical Contrast | Average Acquisition Time (for Entire Chest, Otherwise Stated) | Spatial Resolution/Scan Plane | Temporal Resolution | Scan Parameters | Field Strength |
---|---|---|---|---|---|---|---|
Ventilation | |||||||
2D Steady-state free precession gradient echo–SSFP | FIESTA (GE) TruFISP (Siemens) Balanced FFE (Philips) |
| 3–9 min Free-breathing | Field of view (FOV) = 450 × 450 mm Slice thickness (SL) = 12 mm Matrix = 128 × 128 Coronal or sagittal | 3–4 images/s | Repetition time (TR) = shortest (1.8–2.2 ms) Echo time (TE) = shortest (0.7–0.9 ms) Flip angle (FA) = 65° Bandwidth (BW) = medium–high | 1.5 T |
2D Radiofrequency (RF spoiled gradient echo | SPGR (GE) FLASH (Siemens) FFE (Philips) |
| 3–9 min Free-breathing | FOV = 500 × 500 mm SL = 15 mm Matrix = 256 × 192 Coronal or sagittal | 3–4 images/s | TR = shortest (1.8–2.2 ms) TE = shortest (0.7–0.9 ms) FA = low (<5°) BW = medium–high | 1.5 and 3.0 T |
Inflammation | |||||||
2D Single-shot echo-planar imaging sequence (EPI) | EPI–DWI (GE, Siemens, Philips) |
| 5–7 min Free-breathing | FOV = 420 × 210 mm SL = 6 mm Matrix = 100 × 128 Axial | Low | TR = long (>4000 ms) TE = medium–long (>60 ms) FA = 90°/180° b = 0 and 600 s/mm 2 BW = high Fat suppressed | 1.5 and 3.0 T |
2D Single-shot echo-planar imaging sequence (EPI) | EPI–DWI (GE, Siemens, Philips) |
| 5–7 min Free-breathing | FOV = 420 × 210 mm SL = 6 mm Axial | Low | TR = long (>4000 ms) TE = medium–long (>60 ms) FA = 90°/180° b = 0, 10, 20, 30, 50, 70, 100, 150, 200, 400, 800 s/mm 2 BW = high Fat suppressed | 1.5 and 3.0 T |
2D Fast spin echo | FSE (GE) TSE (Siemens) TSE (Philips) |
| 5–7 min Free-breathing | FOV = 400 mm SL = 5–7 mm Matrix = 256 × 192 Axial and coronal | Low | TR = long (2000–4000 ms) TE = medium–long (>60 ms) FA = 90°/110°–160° BW = low–medium Fat suppression | 1.5 and 3.0 T |
Perfusion/Angiography | |||||||
2D Steady-state free precession gradient echo–SSFP | FIESTA (GE) TruFISP (Siemens) Balanced FFE (Philips) | See scan parameters above | |||||
2D RF spoiled gradient echo | SPGR (GE) FLASH (Siemens) FFE (Philips) | See scan parameters above | |||||
3D RF spoiled gradient echo | SPGR (GE) FLASH (Siemens) FFE (Philips) |
| 12–20 s End-expiratory breath-hold, shallow breathing | FOV = 460 mm Matrix = 40 × 192 × 256 Voxel size (<8 mm 3 ) Sagittal or coronal | Low | TR = short (2.5–3 ms) TE = short (1.0–1.5 ms) FA = 30°–40° BW = medium–high | 1.5 and 3.0 T |
3D RF spoiled gradient echo | TRICKS (GE) TWIST (Siemens) TRACK (Philips) |
| Breath-hold (end-expiratory)/shallow breathing | FOV = 460 mm Matrix = 32 × 96 × 128 Voxel size (<12 mm 3 ) Sagittal or coronal | Medium, 0.5–1 s/volume | TR = short (2.0–2.5 ms) TE = shortest (0.8–1.1 ms) FA = 8°–20° BW = medium–high | 1.5 and 3.0 T |
Structure (including static airways) | |||||||
2D Fast spin echo | PROPELLER (GE) BLADE (Siemens) MultiVane (Philips) |
| End-expiratory with navigator echo triggering 3–7 min according respiratory pace and pattern | FOV = 380–400 mm Matrix = 200 × 200 SL = 5–6 mm Axial and coronal | Low | TR = respiratory rate (2–5 s) TE = short–medium (25–60 ms) FA = 90°/150° ± Fat saturation BW = low–medium | 1.5 and 3.0 T |
2D SSFP | FIESTA (GE) TRUFISP (Siemens) Balanced FFE (Philips) |
| 12–20 s Breath-hold | FOV = 400 mm Matrix = 160 × 160 SL = 2.5–5 mm Axial and coronal | High | TR = shortest (<4 ms) TE = shortest (<2 ms) FA = 40° BW = high | 1.5 T |
3D Fast spin echo | CUBE (GE) SPACE (Siemens) VISTA (Philips) |
| End-expiratory with pencil-beam navigator triggering 5–7 min | FOV = 320 mm Matrix = 160 × 160 SL = 2 mm Sagittal or coronal | Low | TR = respiratory rate (2–5 s) TE = medium (∼60 ms) FA = 90°/variable flip train BW = medium-high Echo train length = 80–140 Fat saturation | 1.5 and 3.0 T |
3D RF spoiled gradient echo | SPGR (GE) VIBE (Siemens) THRIVE (Philips) |
| Breath-hold 10–12 s (inspiratory and expiratory) | FOV = 400 mm Matrix = 200 × 200 SL = 2 mm Isotropic voxel, as low as 8 mm 3 Sagittal or coronal | High | TR = shortest (<1.7 ms) TE = shortest (<0.7 ms) FA = 2° BW = medium–high | 1.5 and 3.0 T |
3D RF spoiled gradient echo | STARVIBE (Siemens) Clinically not available for GE or Philips |
| Free-breathing 3–5 min | FOV = 400 mm Matrix = 320 × 320 SL = 1.2–4 mm Voxel (2–5 mm 3 ) Axial | Low | TR = 7.46 ms TE = 2.46 ms FA = 9° BW = medium | 1.5 and 3.0 T |
3D 2-point Dixon RF spoiled gradient echo | LAVA FLEX (GE) |
| Breath-hold (10s) Free-breathing (pencil-beam navigator) | FOV = 260 mm Matrix = 128 × 128 SL = 3 mm Voxel (<3 mm 3 ) Axial | High | TR = 3.7–4.5 ms TE = min full (<2.0 ms) FA = 2°–10° BW = medium-high | 1.5 and 3.0 T |
3D UTE gradient echo | UTE (GE) PETRA (Siemens) MultiVane (Philips) |
| 7–10 min Free-breathing | FOV = 360 mm Voxel size = 1–8 mm 3 Sagittal or coronal | Low | TR = short (<5 ms) TE = 0.07 ms BW = medium–high | 1.5 and 3.0 T |
3D UTE gradient echo | ZTE (GE) |
| 7–10 min Free-breathing | FOV = 360 mm Matrix = 360 × 360 × 360 Sagittal or coronal | Low | TR = shortest (<1.3 ms) TE = 0 ms BW = medium-high | 1.5 and 3.0 T |
Dynamic Airways | |||||||
2D Steady-state free precession gradient echo–SSFP | FIESTA (GE) TruFISP (Siemens) Balanced FFE (Philips) |
| 3–4 min hyperventilation Coverage larynx to carina ∼ 4 s per slice position | FOV = 450 × 160 mm SL = 8 mm Matrix = 128 × 128 Axial | 4–5 images/s | TR = shortest (1.8–2.4 ms) TE = shortest (<1.2 ms) FA = 35° BW = medium–high | 1.5 T |
3D RF spoiled gradient echo | TRICKS (GE) TWIST (Siemens) 4D TRACK (Philips) |
| 20 s Forced expiration, free-breathing or hyperventilation | FOV = 300 mm Matrix = 80 × 100 SL = 3.2 mm Sagittal | <400 ms/volume | TR = shortest (<2 ms) TE = minimum (<1.1 ms) FA = 2° BW = medium–high | 1.5 and 3.0 T |
Static MR Imaging of the Airways
MR imaging has great potential to assess airways disease. Image quality is comparable with CT, with equal accuracy in central airways measurements. Compared with CT, MR imaging offers better tissue characterization by using different tissue weighting, such as T2 weighting for assessing bronchial wall thickening or proton density weighting for central airways measurements. In a small group of pediatric patients, MR imaging showed similar results to CT and bronchoscopy. Optimal signal-to-noise ratios are usually achieved by using a head and neck coil rather than a standard torso multiphase array coil. High spatial resolution can be obtained both with breath-hold or free-breathing acquisition with isotropic voxel of 1 mm. A summary of the MR imaging sequences for static airways imaging are presented in Table 1 .
Dynamic MR Imaging of the Airways
A key advantage of MR imaging compared with CT is the ability to provide dynamic imaging without exposing the patient to ionizing radiation. Unlike CT, MR imaging dynamic acquisitions can be repeated without increasing the radiation dose. Dynamic acquisition can be 2D and 3D. 2D dynamic airways imaging is usually performed using fast 2D gradient echo sequences, such as spoiled gradient echo or steady-state free precession sequences with temporal resolution in the range of 5 to 10 images per second. 3D dynamic imaging has a lower temporal resolution of between 2 to 3 images per second, but offers the advantage of 3D reformation. Both in pediatric and adult patients a key-hole technique is used to oversample of the central portion of the k-space and achieve a high contrast-to-noise ratio needed to differentiate the trachea lumen from the mediastinum during specific respiratory maneuvers. Several breathing maneuvers can be performed during the dynamic MR imaging examination, including free-breathing, forced expiration, or hyperventilation, which replicate normal breathing conditions or exercise. Sequence settings for 2D and 3D dynamic airways imaging are summarized in Table 1 .
Normal anatomy
Normal Lung MR Imaging Anatomy
In the normal child, the lungs are predominantly filled with air. Because air does not contain protons that produce a signal on MR imaging, the lungs are hypointense on all standard MR imaging sequences ( Fig. 1 ). The pulmonary vessels are visible as linear branching structures within the hypointense air-filled lungs. The pulmonary arteries and veins are isointense to skeletal muscle on T1-weighted images, hyperintense to skeletal muscle on T2-weighted images, and hyperintense to skeletal muscle on contrast-enhanced T1-weighted images with fat suppression. The normal pulmonary fissures are too thin to be well visualized on standard MR sequences, and most often appear as relatively hypovascular bands between lobes. Advanced sequences, such as ZTE and UTE sequences, achieve sub-millimetric voxel resolution that allow visualization of the fissures.
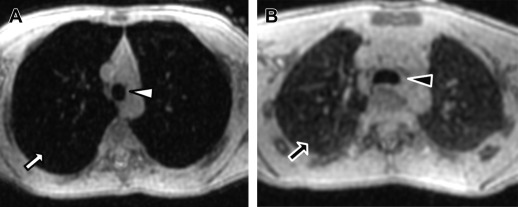
Atelectasis is a common finding in MR imaging, particularly in children receiving sedation or anesthesia. Atelectasis typically appears as a wedge-shaped region of signal hyperintensity within a dependent portion of the lung ( Fig. 2 ). When compared with skeletal muscle, the atelectatic lung is typically isointense on T1-weighted images, hyperintense on T2-weighted images, and hyperintense on contrast-enhanced T1-weighted images with fat saturation. In patients in whom atelectasis obscures a region of interest or mimics pathology, re-imaging in the prone position is often helpful.
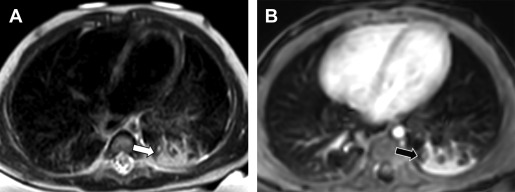
Normal Large Airway MR Imaging Anatomy
The large airways are typically well visualized on MR imaging. Air that fills the trachea and bronchi is hypointense compared with the airway wall and mediastinal structures, serving as a natural contrast agent ( Fig. 3 ). This allows for easy evaluation of the tracheobronchial branching and airway diameter on MR imaging. Airways smaller than 3 mm are typically not visible on standard MR imaging sequences, but the newly developed ZTE sequence is often able to visualize the bronchial tree up to the sixth generation. The wall of the normal airway is thin, and compared with skeletal muscle is isointense on T1-weighted images, slightly hyperintense on T2-weighted images, and slightly hyperintense on contrast-enhanced T1-weighted images with fat suppression.
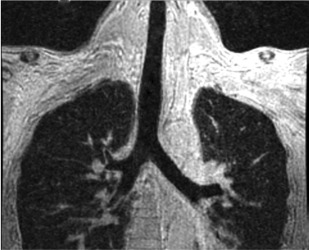
Dynamic MR imaging of the airways in normal children typically shows a round or oval airway at end-inspiration, which decreases in cross-sectional diameter at end-expiration (see Fig. 1 ). Traditionally, a reduction of airway cross-sectional area of less than or equal to 50% during expiration was considered normal, and airway collapse greater than 50% was considered diagnostic of tracheobronchomalacia. However, airway collapse greater than 50% has been shown to be a normal finding in adults, highlighting the limited use of this 50% threshold and the overlap between normal and pathologic collapse. Although degrees of normal airway collapse on MR imaging have not been tested in children, a reduction in cross-sectional diameter of greater than 50% at expiration can also likely be normal, and careful consideration of the patient’s symptoms and other findings is essential when evaluating airway collapse.
Spectrum of MR imaging findings
Congenital Lung Malformations
Congenital lung malformations are characterized by abnormal development of pulmonary parenchyma, vasculature, or both. Currently, lesions are often first detected on prenatal sonogram and further characterized with prenatal MR imaging. After birth, congenital lung malformations are typically characterized and followed with radiographs and CT rather than MR imaging, although the potential role for MR imaging of congenital lung malformations has been described. MR imaging may be considered in newborn patients after feeding and with gentle immobilization, using a feed-and-wrap technique. Generally, if sedation or anesthesia would be needed to perform MR imaging in a young child, CT without sedation would be the preferred modality given concerns regarding the effects of anesthetic medications on neurodevelopment in young children. MR imaging might also be considered in older patients with a congenital lung malformation who may not require anesthesia for MR imaging.
Congenital lung malformations, which may be particularly well characterized on MR imaging include bronchogenic cyst, congenital pulmonary airway malformation (CPAM), pulmonary sequestration, lung hypoplasia, and horseshoe lung (HL) ( Tables 2 and 3 ).
Differential Diagnosis | Diagnostic Criteria | What the Referring Physician Needs to Know |
---|---|---|
Bronchogenic cyst | Congenital foregut duplication cyst arising from the bronchial tree | Cysts may communicate with the airway and become superinfected. MR imaging may show air within a cyst with a thick, irregular, enhancing wall |
Congenital pulmonary airway malformation (CPAM) | Congenital lesions of the lower respiratory tract characterized by cysts, bronchiolar overgrowth and an abnormal bronchial connection | Depending on the type, CPAMs may appear as cystic or solid masses on MR imaging |
Pulmonary sequestration | Nonfunctioning lung tissue that has an abnormal tracheobronchial connection and systemic arterial supply | 2D and 3D angiographic reformations are often helpful for surgical planning and defining anomalous vascular supply and drainage |
Lung hypoplasia | Underdevelopment of the lung due to primary defect or secondary to space-limiting process such as oligohydramnios, skeletal dysplasia, congenital diaphragmatic hernia, or other space-occupying lesion | Lung hypoplasia is most often secondary to a space-occupying lesion, such as in congenital diaphragmatic hernia |
Horseshoe lung | Characterized by an isthmus of lung parenchyma bridging the right and left lungs without and intervening pleural fissure | Horseshoe lung should be considered in any case of lung hypoplasia |
Differential Diagnosis | Pearls, Pitfalls, Variants |
---|---|
Bronchogenic cyst |
|
Congenital pulmonary airway malformation (CPAM) |
|
Pulmonary sequestration |
|
Lung hypoplasia |
|
Horseshoe lung |
|
Bronchogenic cyst
Bronchogenic cysts may be detected in utero, during the newborn period, or later in life. Bronchogenic cysts are most often located within the mediastinum near the trachea or bronchi, although up to 15% can be located within the lung parenchyma. Bronchogenic cysts can cause symptoms due to mass effect or superinfection but are often asymptomatic. Symptomatic lesions are typically treated with surgical resection. Asymptomatic lesions detected in children may be resected given concerns about subsequent development of symptoms and complications. Surgical resection of asymptomatic bronchogenic cysts detected in adulthood is more controversial, although approximately 45% of lesions eventually cause symptoms.
Bronchogenic cysts may first be detected on prenatal ultrasound as an intrathoracic cyst or on chest radiograph as a mass. Cross-sectional imaging is most often indicated for confirmation, characterization, and surgical planning. Contrast-enhanced CT is the most common modality used to evaluate bronchogenic cysts, although lesions are often very well characterized by MR imaging. On MR imaging, bronchogenic cysts are typically thin-walled round or oval structures that contain fluid that is hyperintense on T2-weighted images and variable intensity on T1-weighted images depending on the internal proteinaceous content ( Fig. 4 ). Typically, bronchogenic cysts have a thin wall with mild enhancement on post contrast T1-weigthed images. The wall may become thickened, irregular, and hyper-enhancing in cases of superimposed infection. Bronchogenic cysts may communicate with the airway, in which case they contain air and are at greater risk for superimposed infection.
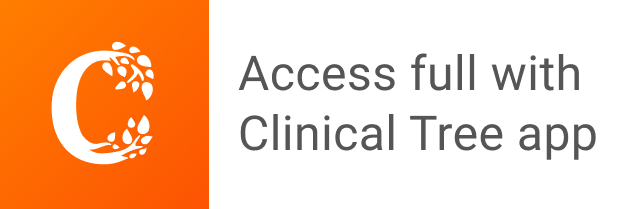