Both term and preterm neonates suffer from diffuse brain injury. Global hypoxic-ischemic injury (HII) describes the diffuse brain injury most common in term neonates. HII is thought to result from decreases in blood flow and oxygen supply. Diffuse white matter injury of prematurity describes the most common diffuse brain injury in preterm neonates. The cause is likely multifactorial. Magnetic resonance (MR) imaging is the most sensitive imaging technique for early diagnosis of brain injury in neonates. This article discusses neonatal diffuse brain injury, the role of MR imaging in predicting neurodevelopmental outcome, and research results using MR imaging techniques.
Hypoxic-ischemic encephalopathy (HIE) describes a clinical syndrome observed in neonates that may be, but is not always, associated with a pattern of global hypoxic-ischemic injury (HII) to the brain on magnetic resonance (MR) imaging; thought to result from decreased blood flow and decreased oxygen supply to the neonatal brain either before, during, or after birth. HII is most likely to occur as a consequence of interruption in placental blood flow and gas exchange, often referred to as asphyxia. The clinical features and extent of injury depend on the severity and duration of the insult, although other factors such as inflammatory changes are also likely to play a role. The incidence of systemic asphyxia is estimated to be 2 to 4/1000 in full-term infants. Antepartum risk factors include maternal hypotension, infertility treatment, and thyroid disease. Intrapartum risk factors include forceps delivery, breech extraction, cord prolapse, abruption placentae, and maternal fever. Postpartum risk factors include severe respiratory distress, sepsis, and shock. Before therapeutic hypothermia, between 20% and 50% of asphyxiated newborn infants with HIE expired during the newborn period and, of the survivors, up to 25% had permanent neurologic handicap including sensory motor deficits and seizures as well as behavioral and cognitive deficits. These statistics were derived before the advent of therapeutic hypothermia in full-term newborn infants with moderate to severe HIE. The results of the latest Cochrane review in 2007 on cooling in newborns with HIE concluded that cooling reduces mortality or disability without increasing major neurodevelopmental disability in survivors. This reduction in death and major disability was reported as significant for severe encephalopathy, but borderline for the moderate subgroup.
The likelihood of diffuse brain injury is higher in preterm infants because of low physiologic cerebral blood flow to white matter, intrinsic vulnerability of premyelinating oligodendrocytes to free radical attack, and also because of an increased frequency of events potentially causing hypoperfusion, such as respiratory distress syndrome, pneumothorax, patent ductus arteriosus, and neonatal sepsis. The initiating mechanisms may be a combination of ischemia and inflammation/infection; these often coexist and can potentiate each other. Major advances in neonatal intensive care have been achieved in the last decades and approximately 85% of premature infants survive in the United States; of the survivors, 5% to 15% have major spastic motor deficits, also known as cerebral palsy, and an additional 25% to 50% have less prominent developmental disabilities, including cognitive and behavioral disabilities.
Extensive human and animal research in the last 30 years has shown that MR imaging is the most sensitive imaging technique for the early detection of diffuse brain injury in term neonates suffering from perinatal HII. MR imaging is also beginning to play a central role in the detection of diffuse white matter injury in preterm neonates. The focus of this article is the global patterns of brain injury seen in HII (profound and partial) typically seen at term and the diffuse white matter injury typically seen in preterm neonates. Focal injuries such as arterial ischemic infarcts or germinal matrix hemorrhages are not discussed.
MR imaging
Transport
MR imaging of a sick neonate is complex. Safe and successful MR imaging of the neonate requires close communication and cooperation between the radiologist, MR technologists, MR nurses, and neonatology staff. In most hospitals, MR imaging suites are far from the neonatology department, necessitating transportation of the compromised neonate. The neonate should be carefully prepared on the floor for safe transportation to the MR unit. The ears must be protected from the loud noise of the MR coils with ear muffs and ear plugs. Heart rate monitoring leads must be changed to MR-compatible leads. A physician and/or nurse experienced in neonatal resuscitation should accompany the infant during the transport and MR procedure, with the infant’s heart rate and oxygen saturation continuously monitored. When ventilated, a respiratory therapist must also be present. Resuscitation equipment appropriate for neonates should be available during the transport and MR procedure.
Special MR-compatible incubators have become available in recent years. The MR-compatible incubator includes a neonatal head coil, MR-compatible monitors, intravenous fluid pumps, ventilators, oxygen tanks, and MR-compatible trolley (eg, Lammers Medical Technology, Lübeck, Germany). The baby is placed into the incubator within the head coil at the neonatal unit, MR-compatible monitors are attached, and then the neonate is transported to the MR suite, imaged, and returned to the floor without being moved out of the incubator and using the same monitors, intravenous lines, ventilator, and so forth. MR-compatible incubators remove the transfers that used to take place in the MR area, making the whole process more efficient for the staff and safer for the neonate.
Sedation
The MR scan takes approximately 30 to 45 minutes and many neonates naturally sleep throughout the scan after being fed, snugly swaddled, and having ear plugs/ear muffs placed. Some need light sedation. Chloral hydrate is used routinely in some centers. The oral form is used, but the rectal form is also available. The oral dose varies between institutions and ranges from 25 mg/kg to 55 mg/kg. Another drug used in neonates for conscious sedation is nembutol, and intravenous or oral forms can be used in adjusted doses. Severely encephalopathic neonates often do not need sedation or may be already sedated by anticonvulsants. In general, sedation is avoided if possible, particularly in the preterm population. All sedation agents have immediate risks such as postsedation apnea and oxygen desaturation with chloral hydrate, which are worse in young and severely injured neonates. Of increasing concern is the potential long-term risk of neurodegeneration with possible cognitive sequelae suggested in animal studies.
Protocol
A field strength of 3 T is optimal for MR imaging and is recommended rather than 1.5 T and lower magnetic field strengths for optimal signal/noise ratio (SNR). A small head coil, preferably a multichannel neonatal head coil that allows image acceleration, is preferred. If not available, a 32-channel adult head coil is the second choice. If only birdcage coils are available, the adult knee coil may be a better alternative, although, given the variability in coil performance, signal to noise comparisons would be needed to confirm the optimal choice. The MR imaging scanning protocol should include a volumetric T1-weighted sequence, a T2-weighted axial sequence, a susceptibility-weighted (SW) image or T2∗ sequence, an axial diffusion-weighted (DW) sequence with a calculated apparent diffusion coefficient (ADC) map, and at least 1 to 2 MR spectroscopy (MRS) samples in the ventrolateral thalamus/lentiform nucleus and centrum semiovale to include the corticospinal tract. For DW sequence, the b values used in pediatric centers range from 700 to 1500 s/mm 2 with higher b values providing better contrast between regions of low and high ADC on DW images. Diffusion tensor (DT) imaging is preferred in advanced centers to allow for tract-specific evaluation of diffusion parameters. Arterial spin labeling (ASL) sequences are becoming commercially available and also show great promise in the evaluation of neonatal brain injury. Resting state functional connectivity (rsFC) is being considered in advanced centers. Although DT imaging with tract-based measures, ASL, and rsFC are promising advanced techniques, their role in diagnosis and prognosis remains unproven.
In daily practice, the MR imaging studies are typically performed at variable times after birth, depending on the severity of the injury, the stability of the neonate, and the availability of scanner time. Therefore, each sequence should be interpreted in reference to its sensitivity at that specific time point and the potential mechanisms of injury. In term neonates presenting with clinical findings of HIE at birth, MR imaging including 1 H-MRS and DT imaging should ideally be performed at less than 6 hours to allow rapid diagnosis and exclusion of risk factors such as hemorrhage before initiation of therapeutic hypothermia. However, rapid MR imaging before hypothermia is difficult and often unethical in practice because neonates transported from outside centers typically arrive hypothermic and MR imaging would delay initiation and therefore potentially decrease the beneficial effects of therapeutic hypothermia. Currently there are only a few centers that have a magnet in the neonatal intensive care unit and scan babies in the first 6 hours; most centers that use therapeutic hypothermia scan the babies while on cooling or after cooling at 4 to 10 days of life. In preterm neonates, particularly those of very low birth weight, transport to MR imaging is also difficult in the first few weeks of life because of the instability and fragility of the neonates. MR-compatible incubators are increasing the safety of these early scans but, in most centers, early MR imaging is not yet deemed clinical and therefore most MR imaging studies in preterm neonates are done near term or as part of research protocols.
Term neonates
Profound HII
Pathophysiology
At the cellular level, the decrease in blood flow and oxygen delivery initiates a cascade of biochemical events. Depletion of oxygen results in a switch to anaerobic metabolism, which is an energy-inefficient state resulting in (1) rapid depletion of high-energy phosphate reserves including adenosine triphosphate, (2) accumulation of lactic acid, and, when severe, (3) the inability to maintain cellular functions. Transcellular ion-pump failure results in the intracellular accumulation of Na + , Ca ++ , and water (cytotoxic edema). The membrane depolarization results in excitatory neurotransmitter release, specifically glutamate from axon terminals, which causes an influx of Na + and Ca ++ into postsynaptic neurons. There is also a decrease in the efflux of Ca ++ across plasma membranes and release from mitochondria and endoplasmic reticulum. The intracellular calcium induces the production of nitric oxide, a free radical that diffuses into adjacent cells that are susceptible to nitric oxide toxicity. Within the cytoplasm, there is an accumulation of free fatty acids secondary to increased membrane phospholipid turnover. When cellular energy failure occurs, acidosis, glutamate release, intracellular Ca ++ accumulation, lipid peroxidation, and nitric oxide neurotoxicity disrupt essential components of the cell and lead to necrosis.
Energy failure and necrosis may occur immediately if there is severe hypoxia and ischemia but, if reperfusion occurs early enough to advert immediate energy failure, delayed energy failure and necrosis may occur. In still milder injuries, delayed apoptosis and delayed autophagosis occur. Thus the severity and duration of the initial hypoxic-ischemic insult likely determine the mode of death; although the most severe injury results in immediate necrosis, milder insult may result in delayed necrosis, apoptosis, or autophagosis. These delayed events require ATP and are associated with activation of a genetic program. Immediate cell necrosis is a passive process of cell swelling, disrupted cytoplasmic organelles, loss of membrane integrity, and eventual lysis of neuronal cells and activation of an inflammatory process. In contrast, delayed cell deaths of any type (necrosis, apoptosis, or autophagosis) are active processes requiring energy and occur in the absence of an inflammatory response. Furthermore, it has been shown that necrosis-apoptosis is a continuum of cell death variants with multiple forms of programmed cell death with different morphologic features and likely distinct molecular mechanisms. It is also known that programmed cell death occurs normally during brain development and these processes might be activated more easily in the immature brain.
Additional genetic, epigenetic, and environmental factors may play a modulating role. For example, genetic mutations resulting in mitochondrial disorders and/or inflammatory processes could increase the vulnerability of neonates to periods of hypoxia and ischemia.
MR imaging features
Specific regions of the deep gray nuclei are extremely vulnerable to damage when the hypoxia-ischemia is profound even though the duration might be short. The resulting MR imaging pattern of injury is called central pattern or thalamus/posterior limb internal capsule pattern. It is equivalent to a cardiac arrest and is seen when there is an acute severe hypoxic-ischemic event such as uterine rupture, abruption of the placenta, cord prolapse, maternal collapse that requires emergent cesarean section, or documented neonatal arrest. These neonates usually present with Sarnat stage II or III encephalopathy, cord pH less than 7.00, first and 5-minute Apgar scores less than 5, need for resuscitation at birth, and seizures. The injury is typically at the ventrolateral thalami and posterior limb internal capsule (PLIC) often with additional involvement of the posterior lateral putamen and the perirolandic cortex. These regions are the most metabolically active and mature areas of the brain with the most advanced myelination with high energy demands. When the insult is more severe or if imaged at a later period in time, the entire thalamus and putamen, the globus pallidi, caudate head, the posterior limb of the internal capsule, hippocampi, corticospinal tracts, dorsal brainstem, and entire cortex may also be affected.
T1-weighted images
No significant signal abnormality or subtle signal change is usually present in the first 2 to 3 days ( Fig. 1 ). If the neonate is scanned between 3 and 7 days of life, increased signal may be observed in bilateral ventrolateral thalami and posterolateral putamina on T1-weighted images ( Fig. 2 ). This signal is graded as mild and the normal hyperintense signal of the PLIC might be preserved. In moderate injury, extension of the T1 hyperintensity anteriorly in the putamen and posteromedially in the thalamus with equivocal or abnormal signal in PLIC is usually seen ( Fig. 3 ). In severe injury, the entire thalamus, putamen and globus pallidi might show hyperintense signal and the normal hyperintense signal of PLIC is typically lost ( Fig. 4 A). Hyperintense T1 signal is also noted in the perirolandic cortex ( Fig. 5 ) and, if the insult is more severe, the insular cortex might be added. In very severe cases, total cortical highlighting is observed, which is a predictor of a poor clinical outcome.
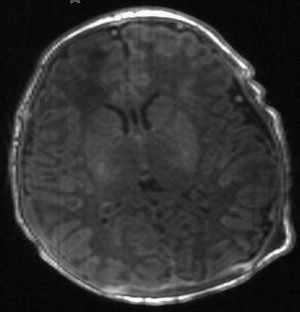
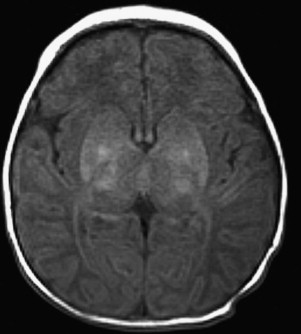
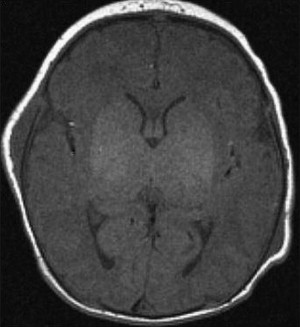
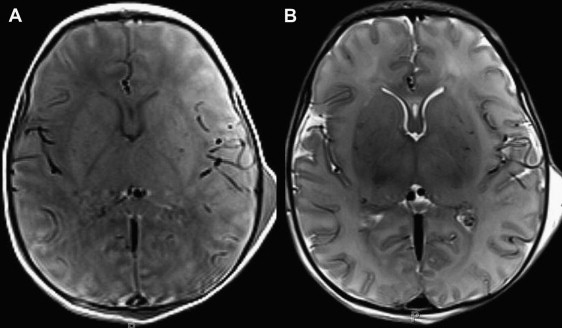
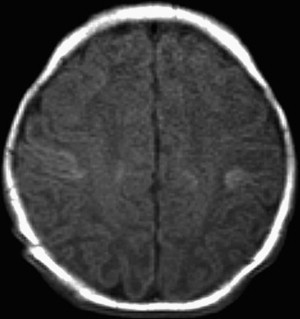
Barkovich and colleagues, in their sequential study of HIE at term infants with DT imaging, MRS, and MR imaging showed that the lesions evolved continuously in the first 2 weeks after birth on all pulse sequences. Thus the milder injury patterns may evolve to more severe injury patterns in the first week as delayed cell death occurs. On T1-weighted images, the hyperintense thalamus/PLIC lesions show a gradual increase in size in the first 7 days and later, in the second week, show smaller, more focal T1 hyperintensity involving the ventrolateral thalami, posterior putamina, and perirolandic cortex. T1 shortening in these areas may persist for several months ( Fig. 6 A).
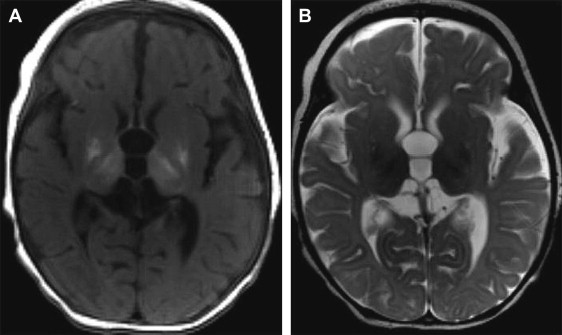
T2-weighted images
T2-weighted images often do not show any signal changes in the first days in central pattern; in some neonates, the deep gray nuclei may become subtly indistinct or the posterior thalami may become isointense with white matter, possibly because of vasogenic edema. If the injury is more pronounced, larger area of thalami, lentiform nuclei, and caudate head may show T2 prolongation and the normal hypointense signal of PLIC may disappear (see Fig. 4 B). After the first 7 days, particularly when the injury is severe, T2 shortening develops in thalami and basal ganglia either in a diffuse or patchy pattern ( Fig. 7 A). The cause of T2 shortening remains controversial. Hemorrhage, calcification, lipid release from myelin breakdown, myelin gliosis, and paramagnetic effects of free radicals are the proposed causes. Hemorrhage is an unlikely cause because T2 shortening occurs several days after the initial T1 changes and these regions do not bloom on gradient echo T2-weighted images or SW images (see Fig. 7 B). T2 shortening may persist for several months (see Fig. 6 B). In the chronic stage, atrophy of the injured structures is seen along with T2 prolongation ( Fig. 8 ).
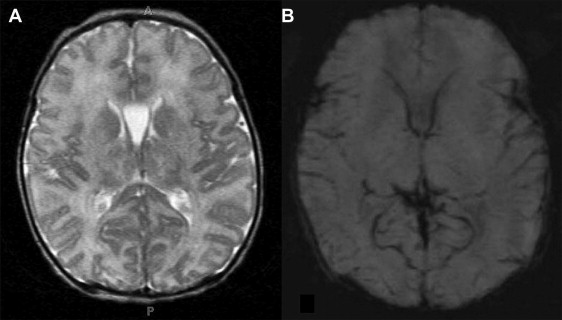
DW imaging
DW imaging enables early demonstration of hypoxic-ischemic brain injury in the first few days when conventional MR sequences show only subtle changes. Diffusion imaging typically shows reduced diffusion in the ventrolateral thalami within the first 24 hours of life; however, because of processes of delayed cell death, the extent of the injury may be underestimated and, rarely, may yield false-negative results at early time points ( Fig. 9 A). It is important to review ADC maps as well as DW images because early injury typically involves regions that are actively myelinating and that are therefore low in T2 signal compared with surrounding brain. Low T2 signal drives the DW imaging signal down, sometimes masking regions of decreased ADC that are readily apparent on ADC maps (see Fig. 9 B). DW images (and ADC values) change continuously during the first week of life, worsening until about day 5 and then pseudonormalizing at around 8 to 10 days ( Fig. 10 ). Regions of the brain with abnormal diffusivity evolve because of delayed cell death, so, for example, ventrolateral thalami may be the only affected area in the first 24 hours but decreased diffusion may become apparent in the posterolateral putamina by day 3 or 4 (see Fig. 9 C, D) and may appear to be affected exclusively by day 8 when the thalamic injury has pseudonormalized. Thus the pattern of abnormal diffusivity varies, presumably because of regional variability in timing of delayed cell death, and the pattern of injury can look different when imaging is performed at different times during this evolution. After pseudonormalization, ADC maps show increased diffusion in the following week secondary to evolving cellular necrosis and vasogenic edema ( Fig. 11 ). Visual analysis of DW imaging may be particularly misleading when there has been widespread injury to the thalamus/PLIC and diffuse white matter/cortex, probably because there is no normal tissue for comparison. A useful clue is to compare the signal of the supratentorial brain with the cerebellar hemispheres, which are typically spared ( Fig. 12 ). In these cases, measuring ADC correctly detects the presence of ischemic tissue.
DT imaging not only provides quantitative information on the brain tissue diffusivity with the ADC values but also information on the relative bias of diffusion directionality that can be assessed with fractional anisotropy (FA). This quantitative information may serve as a biomarker of the degree of tissue injury. Ward and colleagues showed that, during the first week after birth, FA values in the white matter were significantly decreased in neonates with moderate to severe HIE. In severe white matter injury, FA values remained significantly decreased during the second and third weeks after delivery. FA was significantly decreased in the first week in basal ganglia and thalami and became progressively more abnormal within ventrolateral thalami.
1 H-MRS allows identification and quantification of many important brain metabolites. The most frequently studied metabolites are N -acetyl aspartate (NAA), a marker of neurons and their processes; choline (Cho), a marker of membrane turnover; and creatinine (Cr), related to brain energy metabolism. During development, NAA is also found in oligodendrocyte-type-2 astrocyte progenitor cells and immature oligodendrocytes and is therefore an ideal indicator of intact central nervous tissue. Ratios of NAA/Cho and NAA/Cr have therefore been used to assess cellular metabolic integrity in neonatal brain injury.
1 H-MRS can also measure lactate, which occupies a special position in energy metabolism. As an end product of anaerobic glycolysis, the lactate concentration increases whenever the glycolytic rate exceeds the tissue’s capacity to catabolize lactate or export it to the bloodstream. This process takes place during episodes of significant hypoxia or hypoxia-ischemia ( Fig. 13 ). As in HIE, this episode occurs in utero; by the time the neonate has MR imaging, the neonate has been resuscitated and the hypoxia or hypoxia-ischemia has resolved. However, lactate may be detected on these early MR studies, likely because of secondary energy failure and associated with delayed necrosis. This early lactic acidosis is followed by persistently increased lactate levels not associated with acidosis 1 to several weeks after hypoxic-ischemic event. Possible mechanisms leading to persisting cerebral lactic alkalosis are a prolonged change in redox state within neuronal cells, the presence of phagocytic cells, the proliferation of glial cells, or altered buffering mechanisms. This secondary lactate accumulation is accompanied by a decrease in NAA for which the Lac/NAA ratio becomes a good marker of severity of HIE in the subacute to chronic phase.
Animal and human 1 H-MRS studies indicate that glutamate/glutamine (Glx), which is a major excitatory amino acid neurotransmitter in the brain, is also involved in the pathogenesis of HIE. The increased Glx in the extracellular spaces of the brain plays an important role in neuronal injury and death and may also play a role in epileptogenesis and seizure expression induced by hypoxia and ischemia.
Outcome prediction
Data from several studies have shown that the extent of brain injury evident on MR imaging is an important predictor of neurodevelopmental outcome in affected newborns. In addition, the predominant pattern of brain injury as seen on MR imaging was found to be more strongly associated with long-term outcome than the severity of injury in any given region. The thalamus/PLIC pattern of injury on MR imaging predicts severely impaired motor and cognitive outcomes. Children with central pattern of injury are often so severely disabled that they can not be included in long-term follow-up studies. Ability to sit by 2 years of age and walk by 5 years of age occurred in 5 of 7 children who showed involvement restricted to the lentiform nucleus and ventrolateral thalamus, whereas none of the 10 children with more extensive injury, including injury to the perirolandic cortex and hippocampus, were able to reach these goals. Himmelmann and colleagues studied 48 children at a mean age of 9 years (range 4–13 years) with dyskinetic cerebral palsy mostly caused by central gray matter injury and found that most children were Gross Motor Function Classification System level IV (n = 10) and level V (n = 28). The rate of learning disability (n = 35) and epilepsy (n = 30) increased with the severity of the motor disability.
Changes in metabolite ratios were shown to predict long-term neurodevelopment after HIE; better outcomes were associated with higher ratios of NAA to Cho and lower ratios of lactate to choline. Early spectroscopy (18 hours after event) and measurement of high Lac/Cr ratio in 1 H-MRS correlated well with poor neurodevelopmental outcome at 1 year of age. Late-phase lactic alkalosis is also a concern because in vitro studies show that such intracellular alkalosis is detrimental to cell survival after HIE. This information may help to define optimal resuscitation techniques after perinatal HIE to delay rebound brain alkalosis and minimize subsequent cellular injury. Zhu and colleagues recently showed that 1 H-MRS is a useful tool for evaluating the severity and prognosis of HIE noninvasively, and that higher Glx-a/Cr ratio in basal ganglia and thalamus may predict the poor prognosis of neonates with HIE.
Recently Thayyil and colleagues performed a meta-analysis of the published data to reveal the most accurate MR imaging biomarker for prediction of neurodevelopmental outcome after neonatal HIE. The literature from January 1990 to July 2008 was screened in MEDLINE and Embase; to minimize the clinical heterogeneity, preterm infants (born ≤35 weeks’ gestation), healthy control infants, and infants aged 12 months or less at neurodevelopmental testing were excluded from each study. Based on the MR imaging data in this literature, deep gray matter Lac/NAA and Lac/Cr are the most accurate quantitative MR biomarker for prediction of neurodevelopmental outcome after HIE in term neonates.
There has been speculation that treatment with hypothermia delays the appearance of abnormalities on MR imaging but, to date, no study has been able to confirm this. Rutherford and colleagues suggested that, with or without hypothermia, the second week from delivery is the best for maximum lesion detection on conventional imaging. In the recently published TOBY (Total Body Hypothermia) trial, treatment with hypothermia did not influence the ability of posttreatment neonatal MR imaging (median 8 days) to predict neurodevelopmental outcome.
Partial HII
Pathophysiology
The physiologic mechanisms behind these types of injury are not completely understood, but these injuries may be associated with a protracted and difficult delivery or a history of maternal fever. Injury is thought to result from prolonged partial hypoxia-ischemia, although other factors such as inflammatory mediators may play a potentiating role. Neurologic manifestations at birth may be mild and many do not meet the clinical criteria for hypoxic-ischemic encephalopathy. In addition, the onset of neurologic signs can be delayed.
This peripheral pattern of injury seen in these cases of partial HII is characterized by injury to the cortex and the immediately adjacent white matter, with parieto-occipital regions and posterior temporal lobes typically more affected than the anterior regions. One study reported that the precuneus was particularly vulnerable to damage in this pattern of injury. At the cellular level, injury severity is variable, ranging from full-thickness cortical necrosis, laminar necrosis of cortical pyramidal cells, or selective cellular death.
MR imaging features
MR imaging has facilitated identification of these cases compared with ultrasound (US) and computed tomography. Again, the earliest changes are identified on DW imaging within the first 24 hours and can underestimate the extent of the injury at day 2 to 4, but show the pattern of injury with decreased diffusion in peripheral cortex and subcortical white matter ( Fig. 14 ). Findings on DW images might be subtle because of the high T2 signal of the unmyelinated white matter at this age; however, ADC values show approximately 30% to 50% decrease. Follow-up imaging shows a spectrum of outcomes on T2-weighted and T1-weighted images, often involving smaller regions than the initial DW imaging and ADC abnormality, therefore the term stroke or infarct should be avoided when describing these lesions on DW imaging and ADC. Metabolic insult or stress may be used to imply that the tissue has an abnormal DW imaging signal. This region has experienced a severe enough insult to at least alter its energy metabolism and to allow for the possibility that some or all of the cellular elements may recover and that selective cellular death may occur instead of full-thickness injury. On T1-weighted and T2-weighted images, the most obvious finding is the loss of gray-white matter differentiation in affected cerebral cortex and underlying white matter, which can be seen by 2 days. However, the extent of the white matter injury can be underestimated. The final extent of T2 and T1 signal changes in the white matter may become apparent by 4 months but may not be fully appreciable until the end of the first year ( Fig. 15 ). The sagittal and coronal planes are best for detection of these high-convexity lesions ( Fig. 16 ). As the injury evolves, cortical thinning and diminution of the underlying white matter occurs and may result in ulegyri (mushroom-shaped pattern; when injury is in the deeper sulcal portion of the convolutions and spares the crown).
In a prospective study of asphyxiated neonates with 1 H-MRS in the first week of life and correlation with neurologic and developmental status at age 12 months, 1 of the 2 MR spectra was acquired from 5.5 cm 3 of tissue including mostly white matter of the high frontal parasagittal region. This study showed minimal amounts of lactate in this region, particularly in premature infants, without any evidence of asphyxia or other brain damage and it was suggested that the presence of a small amount of lactate in the neonatal brain was not, in itself, evidence of brain injury. Lactate/choline ratios were more strongly associated with neurodevelopmental status and emphasized that lactate had a long T2 relaxation time and was therefore detected and quantitated well on long trapped electron (TE) spectra (TE = 288 ms). High lactate/choline ratio but normal neurodevelopmental status at 12 months was found in 3 patients in this study.
Outcome prediction
These patients may eventually evolve to proximal extremity weakness and spasticity in arms more than legs. Cognitive outcome is variable, but seems worse when the frontal zones are involved; this impairment becomes more apparent as the child matures. Severe motor impairment is uncommon in this group of infants, and they are often considered to have an early normal outcome when seen at 12 to 18 months. Miller and colleagues were first able to recognize cognitive deficits associated with the peripheral pattern of injury at 30 months; more recently they also showed a correlation with verbal intelligence quotient at 4 years of age. Symptomatic parieto-occipital epilepsy may occur later in childhood, often associated with reduced intelligence quotients and visuospatial cognitive functions. In a recent study by Twomey and colleagues, all infants with peripheral or atypical patterns on MR imaging within 10 days of birth had a favorable outcome at 2 years of age compared with those with central patterns. DW imaging predicted outcome group, as did T1-weighted and T2-weighted sequences and cranial US.
de Vries and Jongmans, in their recent review article about long-term outcome after HIE, also emphasized that childhood survivors of HIE in the absence of central patterns are at increased risk of cognitive, behavioral, and memory problems. Although most children with mild HIE studied so far were not found to significantly differ from controls, they were found to be performing between the controls and those with moderate HIE, suggesting a gradual effect. Longitudinal monitoring of educational and behavioral development into childhood and adolescence is recommended in children with both mild and moderate HIE. This recommendation also applies to the infants who participated in the multicenter trials of therapeutic hypothermia.
Term neonates
Profound HII
Pathophysiology
At the cellular level, the decrease in blood flow and oxygen delivery initiates a cascade of biochemical events. Depletion of oxygen results in a switch to anaerobic metabolism, which is an energy-inefficient state resulting in (1) rapid depletion of high-energy phosphate reserves including adenosine triphosphate, (2) accumulation of lactic acid, and, when severe, (3) the inability to maintain cellular functions. Transcellular ion-pump failure results in the intracellular accumulation of Na + , Ca ++ , and water (cytotoxic edema). The membrane depolarization results in excitatory neurotransmitter release, specifically glutamate from axon terminals, which causes an influx of Na + and Ca ++ into postsynaptic neurons. There is also a decrease in the efflux of Ca ++ across plasma membranes and release from mitochondria and endoplasmic reticulum. The intracellular calcium induces the production of nitric oxide, a free radical that diffuses into adjacent cells that are susceptible to nitric oxide toxicity. Within the cytoplasm, there is an accumulation of free fatty acids secondary to increased membrane phospholipid turnover. When cellular energy failure occurs, acidosis, glutamate release, intracellular Ca ++ accumulation, lipid peroxidation, and nitric oxide neurotoxicity disrupt essential components of the cell and lead to necrosis.
Energy failure and necrosis may occur immediately if there is severe hypoxia and ischemia but, if reperfusion occurs early enough to advert immediate energy failure, delayed energy failure and necrosis may occur. In still milder injuries, delayed apoptosis and delayed autophagosis occur. Thus the severity and duration of the initial hypoxic-ischemic insult likely determine the mode of death; although the most severe injury results in immediate necrosis, milder insult may result in delayed necrosis, apoptosis, or autophagosis. These delayed events require ATP and are associated with activation of a genetic program. Immediate cell necrosis is a passive process of cell swelling, disrupted cytoplasmic organelles, loss of membrane integrity, and eventual lysis of neuronal cells and activation of an inflammatory process. In contrast, delayed cell deaths of any type (necrosis, apoptosis, or autophagosis) are active processes requiring energy and occur in the absence of an inflammatory response. Furthermore, it has been shown that necrosis-apoptosis is a continuum of cell death variants with multiple forms of programmed cell death with different morphologic features and likely distinct molecular mechanisms. It is also known that programmed cell death occurs normally during brain development and these processes might be activated more easily in the immature brain.
Additional genetic, epigenetic, and environmental factors may play a modulating role. For example, genetic mutations resulting in mitochondrial disorders and/or inflammatory processes could increase the vulnerability of neonates to periods of hypoxia and ischemia.
MR imaging features
Specific regions of the deep gray nuclei are extremely vulnerable to damage when the hypoxia-ischemia is profound even though the duration might be short. The resulting MR imaging pattern of injury is called central pattern or thalamus/posterior limb internal capsule pattern. It is equivalent to a cardiac arrest and is seen when there is an acute severe hypoxic-ischemic event such as uterine rupture, abruption of the placenta, cord prolapse, maternal collapse that requires emergent cesarean section, or documented neonatal arrest. These neonates usually present with Sarnat stage II or III encephalopathy, cord pH less than 7.00, first and 5-minute Apgar scores less than 5, need for resuscitation at birth, and seizures. The injury is typically at the ventrolateral thalami and posterior limb internal capsule (PLIC) often with additional involvement of the posterior lateral putamen and the perirolandic cortex. These regions are the most metabolically active and mature areas of the brain with the most advanced myelination with high energy demands. When the insult is more severe or if imaged at a later period in time, the entire thalamus and putamen, the globus pallidi, caudate head, the posterior limb of the internal capsule, hippocampi, corticospinal tracts, dorsal brainstem, and entire cortex may also be affected.
T1-weighted images
No significant signal abnormality or subtle signal change is usually present in the first 2 to 3 days ( Fig. 1 ). If the neonate is scanned between 3 and 7 days of life, increased signal may be observed in bilateral ventrolateral thalami and posterolateral putamina on T1-weighted images ( Fig. 2 ). This signal is graded as mild and the normal hyperintense signal of the PLIC might be preserved. In moderate injury, extension of the T1 hyperintensity anteriorly in the putamen and posteromedially in the thalamus with equivocal or abnormal signal in PLIC is usually seen ( Fig. 3 ). In severe injury, the entire thalamus, putamen and globus pallidi might show hyperintense signal and the normal hyperintense signal of PLIC is typically lost ( Fig. 4 A). Hyperintense T1 signal is also noted in the perirolandic cortex ( Fig. 5 ) and, if the insult is more severe, the insular cortex might be added. In very severe cases, total cortical highlighting is observed, which is a predictor of a poor clinical outcome.
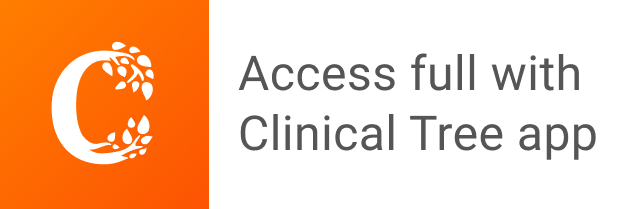