Author (year)
PMID
Number of patients (species)
Organ/cancer
MRI
Ahrar (2011)
21767785
N = 8
Bone tumors (vertebral body, iliac bone, sternum, femoral neck)
1.5 T expanded bore (real time)
Streitparth (2010)
20563575
N = 1
Recurrent osteoid osteoma (right tibial head)
1.0 T open bore (real time)
Sequeiros (2010)
20429757
N = 11
Liver tumors
0.23 T C-arm MRI (near real time)
Kariniemi (2010)
20380606
N = 8
Renal cell carcinoma
0.23 T C-arm MRI (near real time)
Streitparth (2009)
188367767
N = 1
Osteoid osteoma (right fibula)
1.0 T open bore (real time)
Vogl (2008)
18812558
N = 40
Liver metastases
0.5 T (real time)
Carpentier (2008)
18728600
N = 4
Brain metastases
1.5 T (real time)
Ishiwata (2007)
17662349
N = 32
Spine
0.3 T (not quite real time)
Pech (2007)
17509960
N = 66
Colorectal liver metastases
Vogl (2007)
17180325
N = 9
Adrenal metastases
0.5 T (MR thermometry controlled, but CT guided)
Zangos (2007)
16896704
N = 48
Large-sized hepatocellular carcinoma
CT Guided MR Thermometry
Schwarzmaier (2006)
16854549
N = 16
Recurrent glioblastoma multiforme
0.5 T open bore (real time)
Schwarzmaier (2005)
16270287
N = 2
Recurrent glioblastomas
0.5 T open bore (real time)
Vogl (2004)
15675667
N = 1,259
Liver metastases
Unspecified (real time)
Mack (2004)
15459328
N = 232
Liver metastases (breast primary)
0.5 T (real time)
Vogl (2005)
15108016
N = 1
Intrapulmonary chondromas
0.5 T (real time)
Vogl (2004)
14688400
N = 603
Liver metastases (colorectal primary)
0.5 T (real time)
Vogl (2003)
14500854
N = 162
Liver metastases
Puls (2003)
12766895
N = 28
Liver metastases (colorectal, breast, duodenal, liposarcoma primary)
1.5 T (real time)
Segueiros (2003)
12734670
N = 5
Osteoid osteoma
0.23 T open (real time)
Dick (2003)
12623039
N = 125
Live tumors (primary and secondary)
0.5 T open (near real time)
Dick (2003)
12541227
N = 40
Liver, kidney and uterine tumors
0.5 T open (near real time)
Vogl (2002)
12522615
N = 1
Recurrent carcinoid metastasis of the breast
0.2 T open (real time)
Leonardi (2002)
12494354
N = 24
Brain tumors (low-grade glioma, anaplastic gliomas, glioblastomas)
0.5 T open (real time)
Dick (2002)
12460338
N = 9
Malignant renal tumors
0.5 T open (real time)
Vogl (2002)
12409568
N = 899
Malignant liver tumors
0.5 T open (real time) or 1.5 T (real time)
Hindley (2002)
12351555
N = 66
Uterine fibroids
0.5 T open (real time)
Vogl (2001)
11907753
N = 1 (human)
Malignant chondrosarcoma in right infratemporal fossa and left maxillary sinus with orbital invasion
1.5 T (real time/every 30 s)
Mack (2001)
11702139
N = 11
Recurrent extrahepatic abdominal tumors
1.5 T (not specified)
Mack (2001)
11441548
N = 705
Liver metastases
Magnetic field strength not specified (real time)
Leonardi (2001)
11409310
N = 24
Glioma
0.2 T open (cannot find full article and abstract does not explicitly say real time)
Vogl (2001)
11369524
N = 676
Liver metastases and hepatocellular carcinoma
1.5 T or 0.5 T (real time)
Fiedler (2001)
11329194
N = 20
Liver metastases
0.5 T (real time)
Wacker (2001)
11169800
N = 20
Liver metastases
0.2 T open (near real time)
Law (2000)
11042638
N = 12
Uterine fibroids
Magnetic field strength not specified (real time)
Eyrich (2000)
10861702
N = 4 (humans)
Lymphangiomas of the head and neck
0.5 T open (real time)
Law (1999)
10636374
N = 12 (humans)
Uterine fibroids
Magnetic field strength not specified (real time)
De Jode (1999)
10508321
N = 3
Renal tumors
0.5 T open (real time)
De Jode (1999)
10453950
N = 12
Liver tumors
0.5 T open (real time)
Cholewa (1998)
9888320
N = 20
Congenital vascular malformations
Magnetic field strength not specified open (real time)
Vogl (1998)
9807562
N = 127
Liver metastases
Cannot find article, no clues in the abstract
Wacker (1998)
9722861
N = 16
Vascular lesions
0.2 T open (real time)
Kettenbach (1998)
9702896
N = 10
Liver and brain tumors
0.5 T open (real time)
Steiner (1998)
9569329
N = 7
Spine
0.5 T open (real time)
Kahn (1998)
9500275
N = 1
Astrocytoma WHO II
1.5 T (real time)
Schwarzmaier (1998)
9500271
N = 3
Astrocytoma WHO II
1.5 T (real time)
Schoenenberger (1997)
9275913
N = 6 (cadavers)
N = 3 (humans)
Spine
0.5 T open (real time)
Vogl (1997)
9342574
N = 134
Liver metastases
Kahn (1997)
9039620
N = 2
Astrocytoma WHO II
1.5 T (real time)
Feyh (1996)
9467326
N = 5
Head and neck tumors (adenoid cystic carcinoma of the paranasal sinuses, recurrent carcinoma of the tongue and oropharynx)
1.5 T (real time)
Vogl (1995)
7540310
N = 20
Liver metastases
1.5 T (real time)
Blackwell (1993)
10150992
N = 1
Recurrent, metastatic base of skull carcinoma
1.5 T (real time)
Castro (1992)
1370565
N = 1
Right jugulodigastric metastatic squamous carcinoma
0.3 T (real time)
Kahn (1994)
8040431
N = 8
Intracerebral tumors
1.5 T (real time)
Castro (1994)
10151049
N = 43
Head and neck carcinomas
1.5 T (near real time)
The first description of FT for PCa borrowed a term from surgical oncology to describe the procedure—“male lumpectomy” [16].
At present, three major energy sources have been used in focal therapy: cryoablation [16–18], high-intensity focused ultrasound (HIFU) [19], and laser ablation [20]. Other modalities that have been considered suitable and are under investigation are photodynamic therapy and irreversible electroporation.
For FT to be effective, it has to correctly locate and target the cancer within the prostate. There are two main strategies currently deployed to locate and target the tumor: one relies on accurate tissue mapping of the prostate through transperineal-template-based mapping biopsy and the other relies on imaging to identify the clinically threatening index cancer [21].
MR image-guided focal laser ablation (FLA) is dependent on high-quality imaging to locate the cancerous lesion, guiding the ablative modality toward the lesion; monitor the ablation in real time; accurately assess the extent and totality of the ablation posttreatment; and finally might be used to follow up and monitor the prostate in search of a recurrence of cancer in the treated area or the development of new zones.
Although the MR environment poses unique challenges, interventional MR treatments are gaining acceptance. This is due to the fact that MRI with superb ability to discern detailed soft tissue anatomy has high spatial resolution, has the ability to provide functional measurements, and does not have ionizing radiation.
MRI has been used to evaluate prostate anatomy and prostate diseases since 1982 [22].
Laser Ablation
Laser is an acronym for light amplification by stimulated emission of radiation.
The medical field, quick to realize the possibilities in laser energy, was quick to adapt lasers for medical use. Only 3 years after the first commercial laser was built, McGuff et al. reported on the effect of a ruby laser on melanoma cells transplanted in hamsters [23]. Many more studies examining the utilization of laser energy for treating cancer soon followed [24–28].
The process of creating a laser beam involves exciting a particular material (CO2, Nd:YAG, etc.) by an external source (light, electricity, chemical reaction). The material is placed between two mirrors which act as an optical resonator which intensifies the interaction between the electromagnetic field and the excited material (amplification). Making one of the mirrors partly transparent allows the resulting laser beam to exit the resonator.
The characteristics that make laser energy well adjusted to medical implications are the following: it is coherent (the wave trains are exactly in phase), collimated (the beam is parallel), and monochromatic (all the photons have the same wavelength, frequency, and energy).
The physical properties of a laser system are dictated by its wavelength, and the wavelength of the laser is dictated by the source that is being excited, for example, the CO2 laser produces a beam of infrared light with the principal wavelength bands centering around 9.4 and 10.6 μm; the main absorption of biological molecules occurs within the range of wavelength shorter than about 280 nm (ultraviolet). The penetration of light is optimal at wavelengths longer than 1 μm (the near-infrared range of the spectrum). The high water content (60–80%) of most tissue leads to an extensive absorption of infrared radiation and thus to a very efficient energy transfer and heating of the tissue when irradiated with lasers of these wavelengths.
The output of a laser may be a continuous constant-amplitude output (continuous wave) or pulsed. In the pulsed mode of operation, the output of a laser varies with respect to time, typically taking the form of alternating “on” and “off” periods. This application facilitates the depositing of as much energy as possible at a given place in as short time as possible.
The term laser ablation refers to the thermal destruction of tissue by laser. The term “interstitial” laser ablation reflects the fact that the laser fiber is inserted into the tissue as opposed to ablating tissue with a laser while maintaining a buffer medium between the fiber emitting the energy and the tissue being ablated (e.g., air for cutaneous application, saline when applying laser energy in the bladder/ureter).
Interstitial laser ablation was first described by Bown in 1983; he inserted a 400-μm glass fiber into a metastatic skin lesion with the aid of an Nd:YAG laser system and caused local necrosis in the treated area [29].
The basic principles behind laser ablation are the conversion of laser light (excited photons) into heat by tissue. The optical and thermal properties of the tissue as well as the parameters of laser beam influence the extent of the thermal ablation. The optical and thermal properties of the tissue are determined by their structure, water content, and blood circulation. The key concepts are absorption, scattering, reflection, thermal conductivity, and heat capacity.
The prostate as a tissue is suited for FLA due its optical absorption rate without excess vascularity. Highly vascularized tissues such as liver have ample heat conduction therefore limiting the ablation size when performing FLA.
The laser most commonly used for FLA is the Nd:YAG laser, with a wavelength of 1,064 nm, but it is being replaced by more compact and less expensive infrared (800–980 nm) diode lasers. The delivered photons induce an increase in temperature, increasing temperatures to 45 °C and 50 °C, and induce enzymatic changes at the cellular level and an edema develops; temperatures above 60 °C cause rapid coagulative necrosis and instant cell death, but irreversible cell death can also be achieved at lower hyperthermic temperatures (>42 °C), although longer durations are required [30, 31]. Temperatures above 100 °C will cause vaporization of cellular protoplasm, followed by desiccation and shrinkage of the tissue; afterward, any additional laser energy causes a quick temperature rise, and temperatures above 300 °C cause the tissue to burn and carbonization occurs. Carbonization of tissue tends to occur closer to the laser fiber and decreases the optical penetration and heat conduction of the tissue being ablated. It limits the size of lesion produced and due to the irregular and unpredictable light absorption in the tissue causes unpredictable ablation volume; therefore, during FLA, it is advisable to refrain from heating above 100 °C.
Many new modifications have been made to allow for efficient tissue ablation. The introduction of the flexible laser fiber, utilizing quartz fibers of small diameter (250–1,000 μm), allows the application of FLA through flexible fiber-optic devices and through thin needles [32, 33].
Interstitial fibers, which are quartz fibers that have flat or cylindrical diffusing tips and are 10–40 mm long, provide a much larger ablative area of up to 50 mm [34, 35].
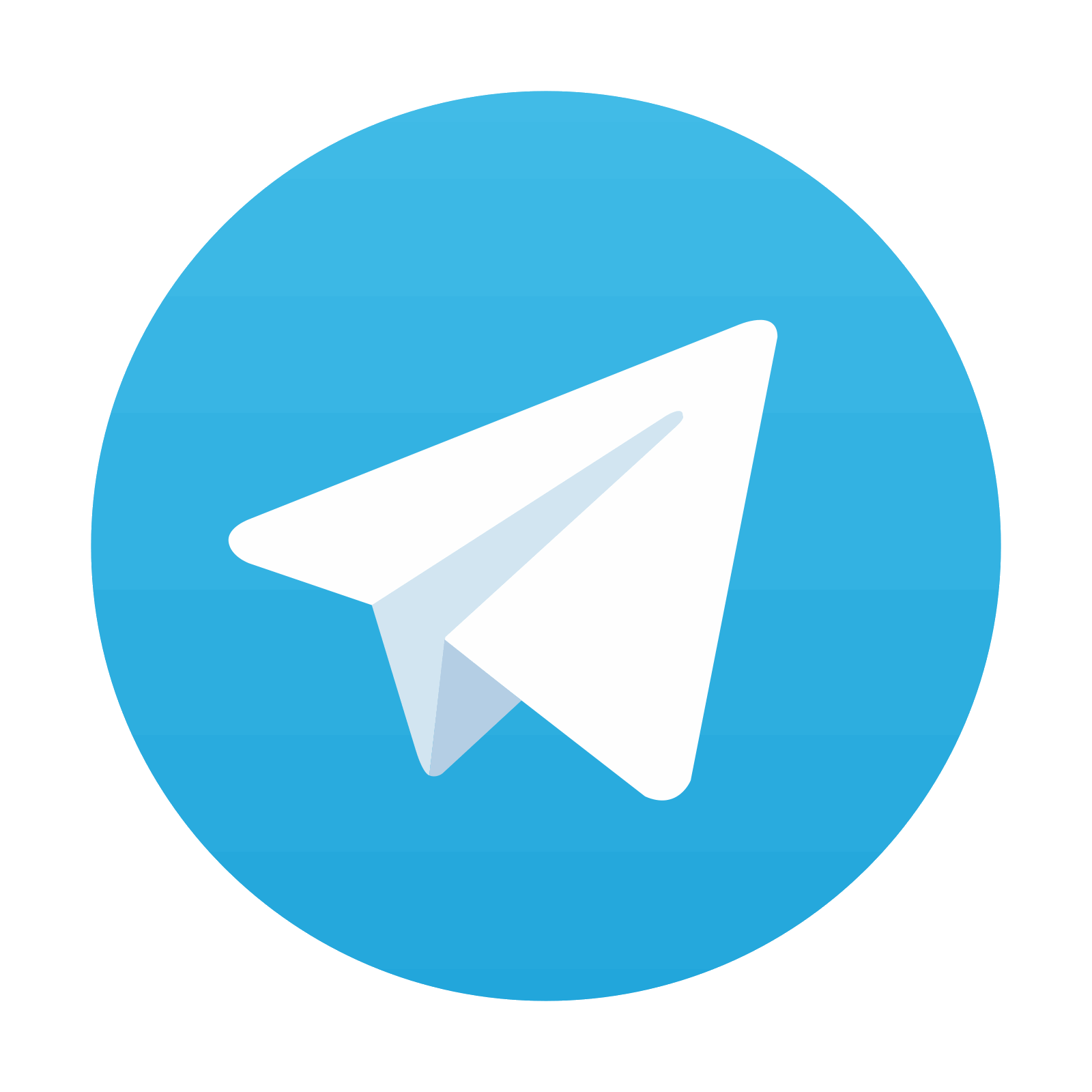
Stay updated, free articles. Join our Telegram channel
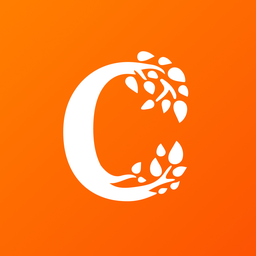
Full access? Get Clinical Tree
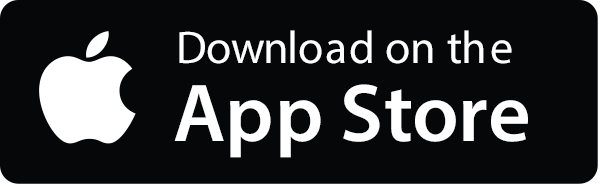
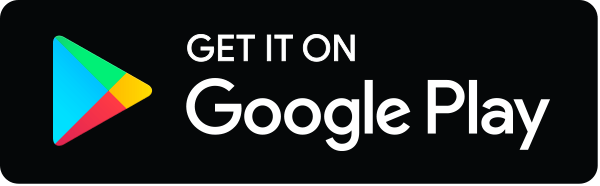