Fig. 2.1
Rapid advancements in photon therapy in the 1980s and 1990s made photon therapy competitive with particle therapy. Comparable progress is needed in particle therapy to realize its highest potential
The following sections describe the rationale for particle therapy, the current state of the art, and its limitations and summarize ongoing and planned research to advance it. While most of the discussion is on protons, issues related to carbon therapy and the potential of other light ions are also addressed.
2.2 Rationale for Charged Particle Therapy
As charged particles traverse a medium (e.g., a patient or a water phantom), they scatter and lose energy continuously. The rate of energy loss per unit distance traveled, called “linear energy transfer,” or LET, increases as particles slow down until their energy is fully depleted and they come to a sudden stop. The shape of the resulting depth dose curve, called the Bragg curve, is illustrated in Fig. 2.2. A lower dose is deposited at points at the entrance and in the plateau region, i.e., in the region before the steep rise in dose, and most of the dose is deposited in a region around the Bragg peak. Because of the statistical nature of the scattering and energy loss processes, there is straggling of the range of the particles, and, therefore, the steepness of the distal fall-off of dose is somewhat moderated, the magnitude of which depends on the initial energy. The depth of maximum penetration also depends on the initial particle energy. This type of dose deposition pattern does not lend itself to treating finite-size, arbitrarily shaped tumors. Therefore, the incident beam is spread out longitudinally and laterally, using scatterers and range modulators, and shaped, using apertures and compensators, to produce a volume of high dose that conforms to the shape of the target. Alternatively, pencil beams (beamlets) of a sequence of energies and varying intensities are scanned to produce a pattern of conformal dose distribution that optimally balances tumor dose against normal tissue sparing. These two modes of treatment planning and delivery are called passively scattered particle therapy (PSPT) and intensity-modulated particle therapy (IMPT).
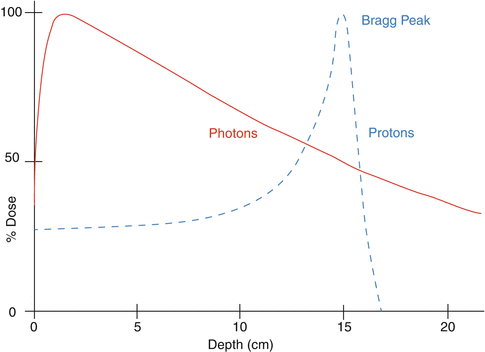
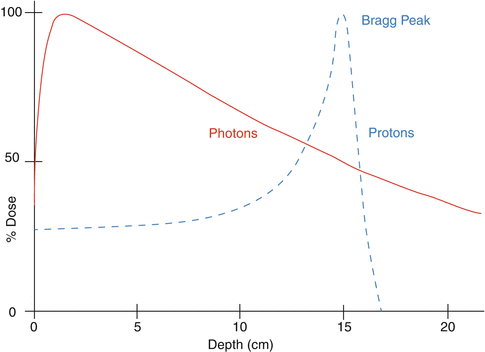
Fig. 2.2
Typical depth dose characteristics of protons and photons in water
In contrast, photons deposit the largest amount of dose near the entrance and continue to deposit significant dose beyond the target (Fig. 2.2). In short, the rationale for particle therapy is that it deposits low dose proximal to the tumor target and virtually no dose beyond the target. Heavier ions have similar characteristics except that their interactions with the media may result in a low-dose fragmentation tail of lower atomic number particles distal to the end of the range of the primary particles.
The physical characteristics of particles can be used to produce dose distributions that appear to be exquisitely superior compared to those possible with photons. Examples of medulloblastoma, nasopharynx and base of skull [1] are shown in Fig. 2.3. The potential of particle therapy over photons and the potential of carbon ions over both protons and photons are, in principle, evident based on physical dose distributions.
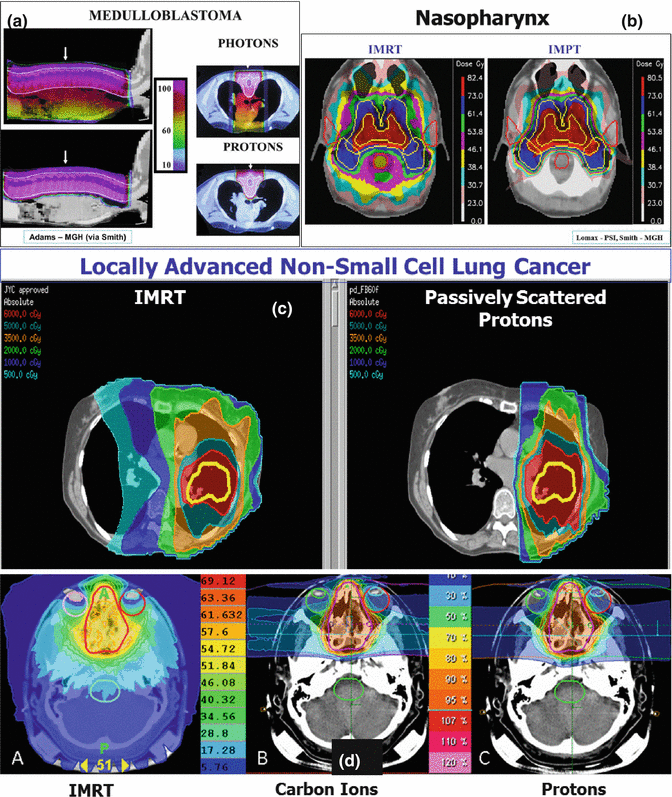
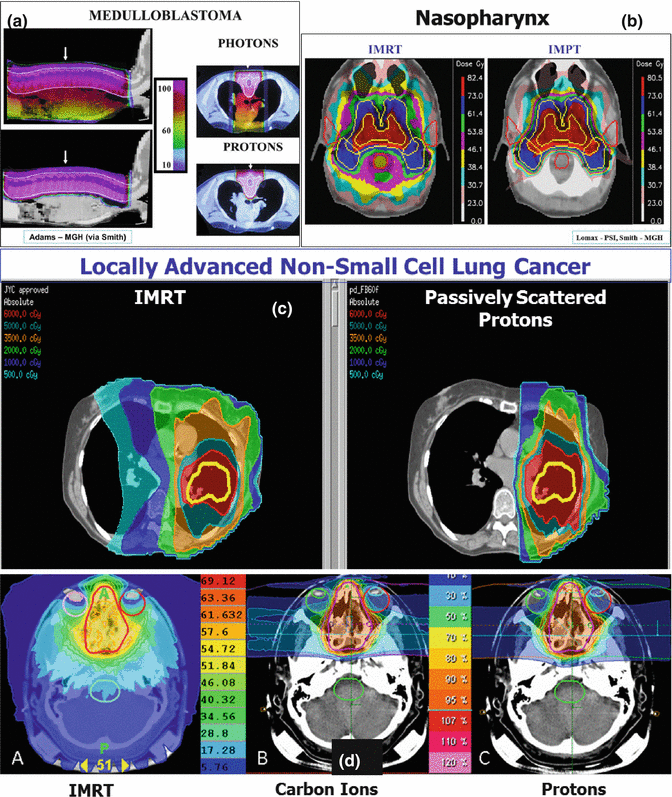
Fig. 2.3
In addition to their physical characteristics, the biological effectiveness of particles must also be considered. For protons, the relative biological effectiveness (RBE), based on the average of multiple experiments, has been assumed to have a constant value of 1.1 for all cancers and normal tissues. This is an approximation that could have unforeseen consequences, and more accurate determination of RBE and its proper incorporation into proton treatment plan design and evaluation may lead to further enhancement of the effectiveness of protons. For heavier ions, LET is considerably higher than that of protons, produces more complex DNA double-strand breaks, and, therefore, leads to much higher RBE. Furthermore, it can no longer be approximated by a constant value. Heavier ions also have a lower oxygen-enhancement ratio (OER). Both the higher RBE and the lower OER, especially in and around the Bragg peak, can be a considerable advantage in tumor cell killing, particularly for resistant tumor cells. This is the primary rationale for using heavier ions for cancer treatments.
Figure 2.4 shows LET and dose as a function of depth for three different particles, and Fig. 2.5 shows RBE-weighted depth doses for protons and carbon and RBE and OER as a function of LET for alpha particles. Note that even for protons the RBE varies.
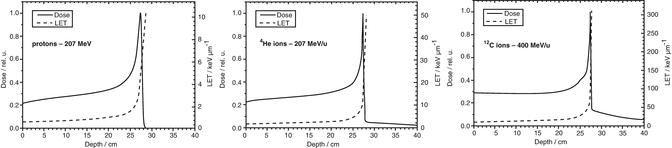
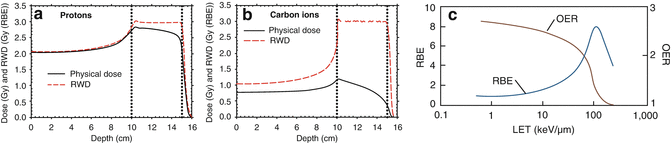
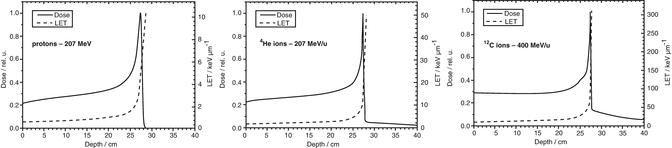
Fig. 2.4
Depth-dose and depth-LET curves for 20 cm radius fields of protons, 4He and 12C, all with a range of ~28 cm (Guan—unpublished)
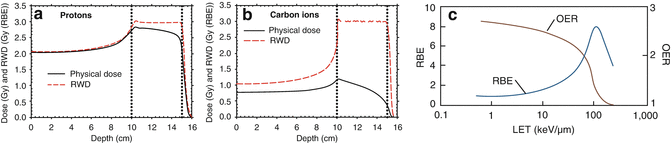
Fig. 2.5
(a, b) Physical doses for proton and carbon ion SOBP required to yield a constant RBE-weighted dose (RWD) of 3 Gy (RBE) are shown. Each SOBP consists of pristine Bragg peaks with fluences optimized to yield a constant RWD [3]. (c) RBE and OER as functions of LET for T1 kidney cells irradiated with alpha particles and deuterons [4]
2.3 Current Status
Although particle therapy has been used for many decades, the number of patients treated with it is considerably smaller than with photons. Because of the recent dramatic increase in the number of facilities and the associated high cost, questions have been raised about the efficacy, cost effectiveness, and the motivation of establishing such facilities. The following are some examples.
Brada et al. performed a systematic review and analysis of published clinical results of proton therapy in 2007 and “found no convincing evidence that protons [implicitly, particles in general] are superior to photons [5].” Five years later, De Ruysscher et al. updated the findings of Brada et al. and confirmed that Brada’s conclusions still stand and that “except for rare indications such as childhood cancer, the gain from introducing proton therapies into clinical practice remains controversial [6].”
Similarly, ASTRO’s Emerging Technology Committee published an evidence-based review of proton beam therapy and found that there was no evidence to date to recommend proton beam therapy for the lung, head and neck, GI, and non-CNS pediatric malignancies [7]. In some cases (hepatocellular carcinoma and prostate), protons have been found to be effective but not superior, and in other cases (e.g., CNS), there is insufficient data to draw conclusions. However, there is evidence of the benefit with protons for ocular melanomas and chordomas.
Recently, there have been reports of additional positive experiences based on small studies. For instance, Chang et al.’s phase 2 study of high-dose proton therapy with concurrent chemotherapy for unresectable stage III non-small cell lung cancer, which involved 44 patients, reported an impressive median survival of 29 months, grade 3 pneumonitis of 3 %, and grade 5 esophagitis of 12 % [8]. Another example, from a presentation by Frank at the ASTRO 2013 Annual Meeting, reported a considerably reduced incidence and duration of the use of feeding tube for IMPT compared to IMRT for oropharyngeal cancers.
However, until recently, there have been no randomized trials and no high-level systematic large-scale studies directly comparing protons and photons. Thus, the overall evidence can at best be considered low level and mixed. Weak evidence is not just limited to protons but is also true for carbon ion therapy. Debus of DKFZ during his presentation at the PTCOG 52 meeting in 2013 concluded that, while there are clinical data from prospective phase I/II and phase II trials that support the hypothesis that there is a role of carbon ions in oncology, evidence of clear superiority is lacking.
There is also a lively debate about the socioeconomic aspects of proton therapy. For instance, at the 2007 ASTRO Annual Meeting, Tim Williams, then president of ASTRO, wondered about the reasons (motives) for establishing proton therapy centers. His list included belief in clinical efficacy, program differentiator, revenue generation, institutional prestige, and defensive maneuver. Unfortunately, motives are not always altruistic. Even the belief in clinical efficacy is, in general, based on the dose distribution characteristics of protons and on treatment planning studies rather than on clinical data.
An obvious question is “Why has the clear advantage of particles on paper not translated into clinical practice?” There may be multiple factors including immature technology or limited experience up to now; the greater vulnerability of particles to uncertainties such as interfractional changes, intra-fractional motion, and setup variability; the accuracy of computed dose distributions; uncertainty in relative biological effectiveness (RBE), etc. Some of the limitations of particle therapy and concerns about them have been apparent for several decades. For instance, Goitein et al., realizing the vulnerability of protons to uncertainties, suggested various means for accounting for them [9–12]. Even in the face of such uncertainties, the gap between protons and photons at that time was large enough that protons could safely be assumed to be superior. However, with the major advances in photon therapy over the years, that is no longer the case. Furthermore, due to the availability of advanced and sophisticated imaging and treatment planning tools, research is revealing the consequences of the impact of uncertainties on particle therapy. The following subsections give some illustrative examples.
2.3.1 Interfractional Variations
Figure 2.6 illustrates the greater vulnerability of proton dose distributions to interfractional anatomic variations compared to IMRT. After 2 weeks of radiotherapy, the tumor volume has cavitated considerably. For IMRT, the application of the original beam and intensity configuration to the CT image 2 weeks later produces virtually the same dose distributions. For protons, however, the loss of tumor tissue allows protons to penetrate much further. Accommodation of such dose perturbations requires frequent repeat CT imaging, evaluation of anatomy changes on dose distribution, and adaptive replanning, if indicated.
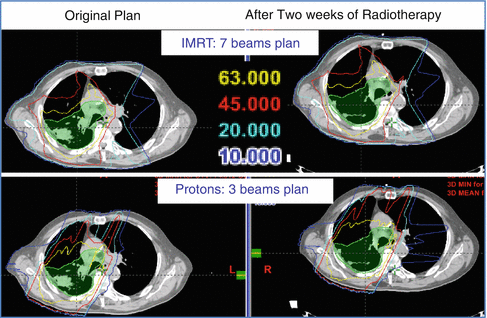
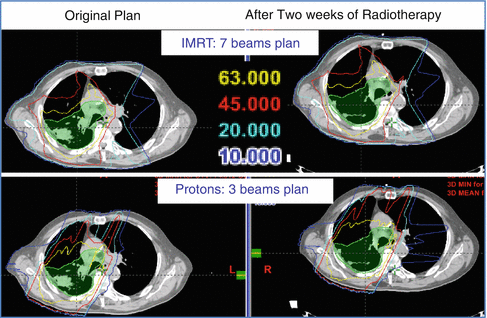
Fig. 2.6
Illustration of the greater impact of tumor shrinkage over the course of treatments on proton dose distributions than on IMRT dose distributions
2.3.2 Respiratory Motion
Figure 2.7 is an example of the impact of the respiratory motion of the tumor on proton dose distributions. The proton dose distributions shown on the left were designed using an average of a free-breathing CT. The green, orange, and red structures are the motion-incorporated internal target (ITV), clinical target (CTV), and gross target (GTV) volumes. The prescribed dose is indicated by the yellow isodose line and assumed to correspond to the dose expected to be delivered. The right panel shows that the dose distribution in the maximum inhale phase is quite different from the one used to make the treatment decision.
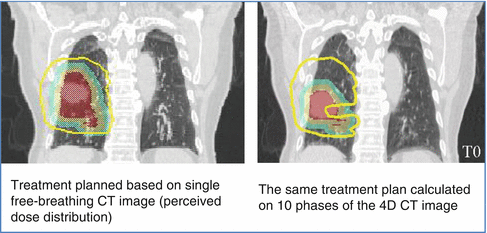
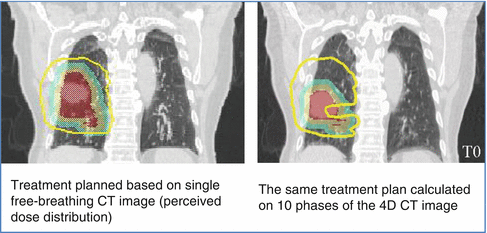
Fig. 2.7
The impact of respiratory motion of the lung tumor on the proton dose distribution. It should be pointed out that it is not just the motion of the tumor that needs to be considered but the motion of any portion of the anatomy in the path of the protons
2.3.3 Relative Biological Effectiveness
There are important gaps in our knowledge of the biological effectiveness of particles, including protons. While the following discussion is focused on protons, uncertainties in relative biological effectiveness (RBE) of any of the particles would have unwanted consequences.
There is evidence that the (RBE) of protons is a complex function of numerous factors including depth of penetration (implicitly on LET), dose per fraction, total dose, cell type, oxygenation, biological end point, etc. [13–16]. Yet, in current practice, RBE is simplistically assumed to be 1.1 in all situations [17, 18]. This assumption may lead to an increased risk of injury to surrounding normal tissues where RBE may be higher than 1.1 or to recurrences in tumors where RBE may be less than 1.1. Moreover, the opportunity to explicitly take advantage of the higher RBE to achieve greater killing of tumor cells is not realized.
The claim is often made that there is no clinical evidence to suggest that the assumption of RBE of 1.1 has caused any harm. To date majority of clinical proton treatments have employed PSPT [19]. This, along with statistical uncertainties from the limited clinical data, has likely obscured the importance of RBE variability.
Another rationale sometimes given in support of the continued use of proton RBE of 1.1 is that the high RBE is confined to a very narrow region from just before the Bragg peak to the end of distal fall-off. Because of the very rapid fall-off of dose, the increased RBE is, therefore, biologically inconsequential. However, the distal edge is often degraded by the passage of beams through tissues, especially through complex heterogeneities, and may extend over a significant volume.
Furthermore, IMPT dose distributions contributed by individual beams are highly heterogeneous. This means that the biologically effective dose delivered may be substantially different from the dose based on RBE of 1.1. On the other hand, the inherent flexibility of IMPT offers the opportunity to capitalize on variable RBE through the incorporation of such information into the treatment optimization process. Higher LET protons have a higher biologically effectiveness for the same dose deposited. Thus, for the same dose deposited, one can use a smaller number of the high LET protons or a larger number of lower LET protons. This concept can be used to confine high RBE portions of beamlets into the target volume and away from critical normal structures, producing more effective treatments.
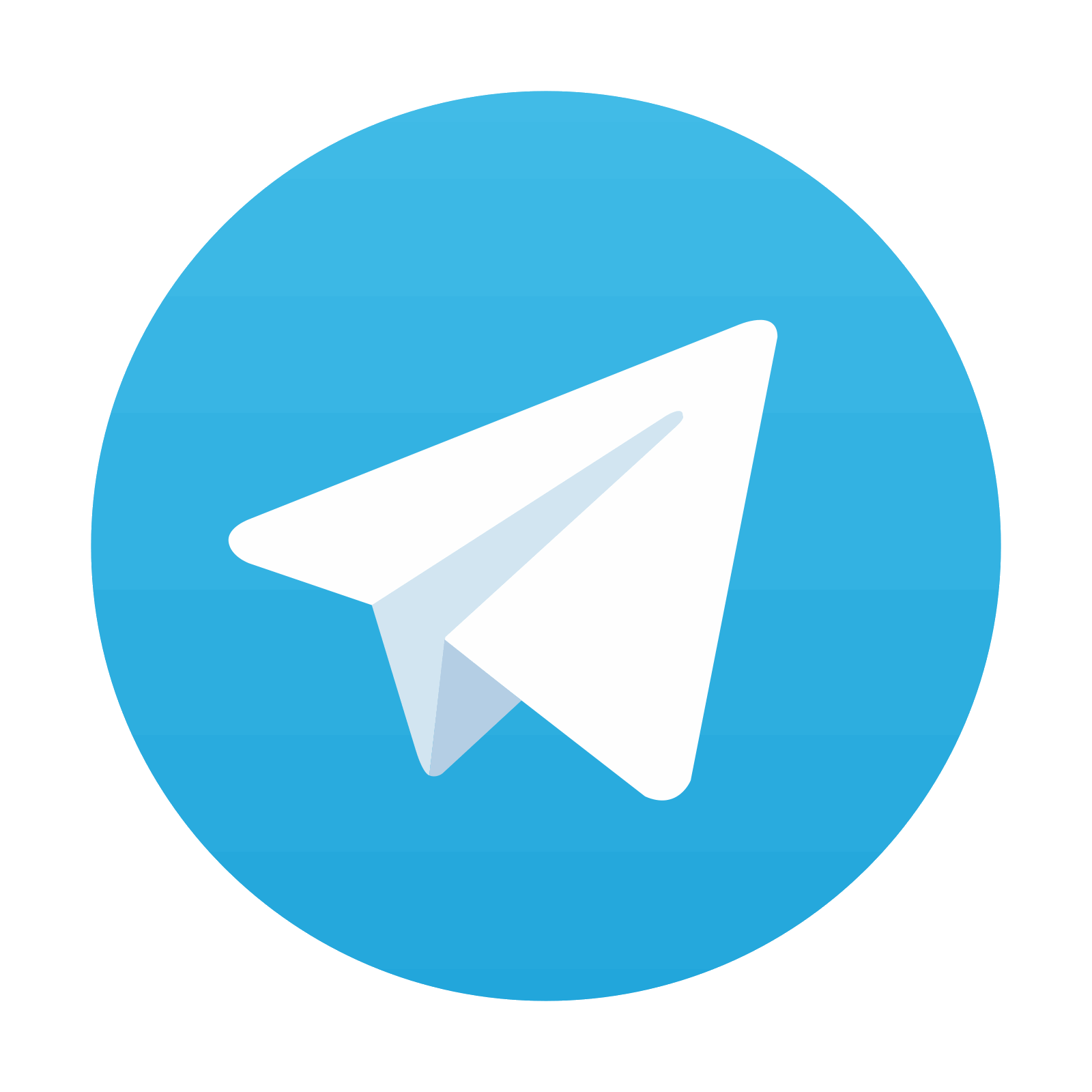
Stay updated, free articles. Join our Telegram channel
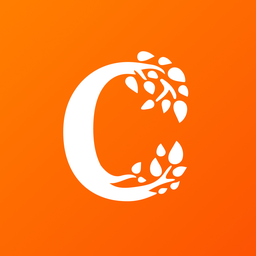
Full access? Get Clinical Tree
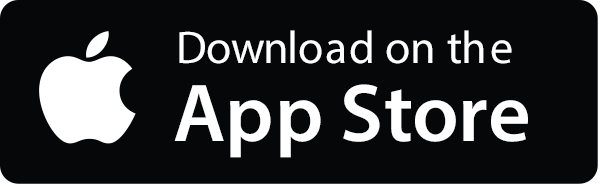
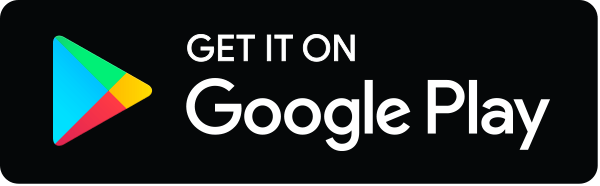