Fig. 1.1
Leukocyte adhesion cascade. Leukocytes are attracted by chemokines and recruited by activated endothelial cells. Leukocyte rolling is mediated by selectins, activation is mediated by chemokines, and arrest is mediated by integrins. Additional adhesion molecules like ESAM (endothelial cell-selective adhesion molecule), JAM (junctional adhesion molecule), cadherins, and PI3K signaling allow leukocytes firm adhesion and ultimately the transmigration of leukocytes through the endothelial layer (Adapted from Ley et al. [12])
In the early phases of atherosclerosis, monocytes enter the arterial intima and differentiate into macrophages. Recent work has demonstrated that hyperlipidemia increases a subpopulation of particularly proinflammatory monocytes in blood that preferentially home to nascent plaques. These proinflammatory monocytes, which are identified in mice by high expression of the surface marker Ly6C and low expression of CD43 (Ly6Chigh CD43low), produce high levels of atherogenic signaling molecules [13–17]. It is currently unclear whether similar subpopulations of atherogenic monocytes exits in humans; however, recent data suggest that mon1 monocyte subsets found in spleen and atherosclerotic lesions (CD14++ CD16- CCR2+ CX3CR1lo; CD62L+ CD115+) are functionally similar to proatherogenic murine Ly6Chigh CD43low cells. More investigation will be required to refine our understanding of the role of monocyte heterogeneity in human atherosclerotic disease.
Following infiltration into the vascular wall, monocytes differentiate into macrophages, which predominantly have an M1 proinflammatory phenotype [18, 19]. These classically activated macrophages develop in response to the inflammatory cytokines tumor necrosis factor-α (TNF-α), interferon-γ (IFN-γ), and Toll-like receptor (TLR) agonists. Plaque-resident macrophages produce inflammatory mediators, which direct recruitment of additional immune cells into the evolving atherosclerotic plaque. Macrophage differentiation is marked by an increased expression of scavenger receptors (SRs), such as scavenger receptor-A (SR-A (CD204)), CD36, macrophage receptor (MARCO), and lectin-like oxidized LDL receptor (LOX-1), which mediate macrophage internalization of LDL particles and apoptotic bodies. Macrophages also engulf cholesterol particles by micropinocytosis of native LDL and phagocytosis of aggregated LDL [20, 21]. In the setting of hyperlipidemia, intimal cholesterol deposition overwhelms the ability of monocytes/macrophages to clear these noxious molecules, and lipid-overloaded macrophages turn into “foam cells,” which contain abundant cholesteryl ester droplets within the cytosol. Foam cell formation is the hallmark feature of fatty streaks which are the earliest stage of an atherosclerotic plaque. Foam cells have a highly inflamed phenotype, constitutively active NFkB inflammation pathways, and produce atherogenic cytokines and matrix metalloproteinases (MMP) [22].
1.3 Plaque Progression
As atheroma formation progresses, lipid-engorged foam cells undergo apoptosis, and the buildup of apoptotic bodies and necrotic debris results in the formation of a “necrotic” plaque core (Fig. 1.2). Inflammatory mediators stimulate plaque expression of the prothrombotic clotting protein tissue factor (TF) on cells within the plaque [23, 24].
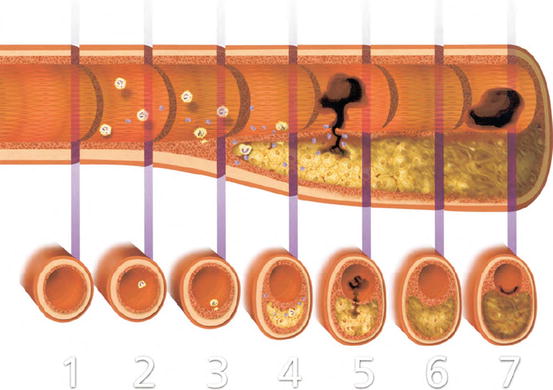
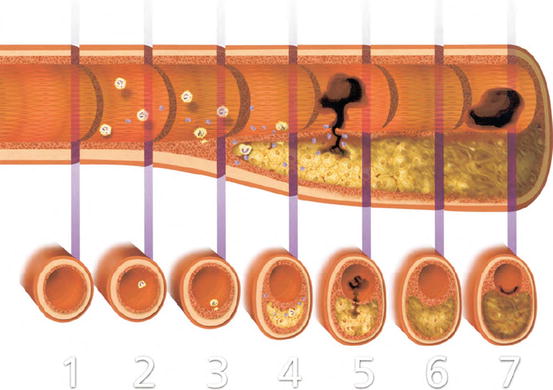
Fig. 1.2
Development and complications of atherosclerotic plaques. Illustration of longitudinal and transversal sections of an artery from the initiation of the lesion (1) to the thrombotic complications of atherosclerosis (5–7). In the early phase of plaque formation (2), dysfunctional endothelium induces an increased vascular permeability allowing the progressive accumulation of lipids in the artery wall. Dysfunctional endothelial cells recruit inflammatory leukocytes by releasing chemokines and expressing adhesion molecules. (3) Accumulating macrophages in the lesion engulf modified lipoproteins and form lipid-laden foam cells. Leukocytes and vascular cells release inflammatory cytokines that sustain and amplify the recruitment of inflammatory cells within the intima and promote smooth muscle cell migration and proliferation. (4) Progressive accumulation of lipids and necrotic cells forms an acellular “necrotic core” in the lesion, overlapped by a fibrous cap, composed by extracellular matrix and smooth muscle cells. Inflammation further increases the production of prothrombotic molecules and proteinases that can cleave extracellular matrix proteins of the fibrous cap and weaken its resistance from rupture. (5) When the fibrous cap ultimately breaks, coagulation factors in blood gain access to prothrombotic molecules in the necrotic lipid-rich core, forming a thrombus. If fibrinolytic mechanisms fail to overcome clot formation, an occlusive thrombus may form and provoke an acute coronary syndrome. (6) Plaques can evolve towards a more fibrous lesion, rich in extracellular matrix and smooth muscle cells, and often calcified nodules. These more “stable” lesions can however become significantly stenotic. (7) Superficial erosion can occur in advanced lesions. Erosion is characterized by a local desquamation of endothelial cells, exposing procoagulant signals, and associates with the formation of a mural thrombus without any visible signs of plaque rupture (Adapted from Libby [10])
The proinflammatory milieu within the nascent plaque stimulates migration and proliferation of smooth muscle cells (SMC) in the burgeoning atheroma. Cytokines such as transforming growth factor-β (TGF-β), which are released by vascular and immune cells, promote SMC proliferation and drive a phenotypic switch from a quiescent SMC contractile form to a proliferative and hypersynthetic phenotype. The accumulation of these SMCs in the plaque is associated with the production of several extracellular matrix (ECM) proteins (i.e., collagen, elastin), which increase plaque mass. Importantly, the plaque ECM components also create a scaffold that provides tensile strength to the atheromatous lesion [25]. In addition to ECM deposition, impaired macrophage clearance of apoptotic debris (impaired efferocytosis) also promotes plaque expansion.
Curiously, plaques often enlarge outward (outward or positive remodeling), preserving the lumen until later phases of the disease when the direction of plaque expansion shifts inward to obstruct blood flow by impinging on the vessel lumen. In this manner, the clinical manifestations of atherosclerosis often only become apparent in the later phase of the disease when luminal obstruction and arterial insufficiency result in tissue ischemia (Fig. 1.2).
As the expanding plaque outstrips its oxygen supply, the artery wall adventitial vasa vasorum sprout new blood vessels that penetrate the media and intima to augment plaque oxygen delivery. Neoangiogenic processes may attenuate cell death and necrotic core accumulation by providing nutrients and oxygen to cells deep within the plaque. Neoangiogenic blood vessel also provides a pathway for the entry of additional immune cells into the center of inflamed plaques [26].
As the plaque progresses, the necrotic core remains covered by a fibrous cap that supports the luminal endothelial cells. This fibrous cap critically maintains blood vessel integrity and prevents exposure of the prothrombotic atheroma core to the circulating blood pool. In the setting of ongoing inflammation, cells within the atheroma (primarily macrophages, ECs, and SMCs) produce enzymes that degrade arterial ECM proteins to enable geometrical remodeling. These enzymes, which include MMPs and cathepsins, directly breakdown interstitial fibrillar collagen and elastin which provide tensile strength to the plaque fibrous cap [27, 28].
1.4 Plaque Complications
The complications of atherosclerotic plaques are largely related to adverse effects on delivery oxygen and nutrients to tissues. In cases where inward remodeling progressively results in obstruction of blood flow, patients experience ischemic symptoms caused by arterial insufficiency. In the insidious setting of stable plaque progression, the symptoms of tissue ischemia often develop gradually and may be prompted by activities which increase utilization and oxygen demand of the affected organ. Symptomatic atherosclerotic complications also occur acutely, when a previously asymptomatic atherosclerotic plaque causes sudden arterial thrombosis and tissue ischemia. Indeed, it is estimated that thrombosis of subclinical atherosclerotic plaques accounts for the majority of fatal myocardial infarctions annually [29]. Subclinical and clinical plaque-associated thrombosis and healing is also a common mechanism for plaque expansion that promotes inward remodeling and narrowing of the arterial lumen [30]. The three main types of acute plaque complications discussed below are plaque rupture, intraplaque hemorrhage, and plaque erosion.
1.4.1 Plaque Rupture
Plaque rupture is the most common form of atherosclerotic plaque complications, and it accounts for ~70 % of fatal myocardial infarcts (MI) [31]. Plaque rupture occurs when shear forces of flowing blood or microcalcification impurities cause physical rupture of the fibrous cap that underlies the endothelium and separates the prothrombotic atheroma core from luminal blood. Exposure of the TF-rich plaque core to flowing blood results in clotting cascade activation, platelet deposition, and thrombus formation that can acutely obstruct arterial blood flow.
Importantly, rupture prone or “vulnerable atherosclerotic plaques” have characteristic features that include thin fibrous caps (<65–55 um), a large lipid-rich core (>40 % of plaque’s volume), and active inflammation as evidenced by extensive accumulation of macrophages [32]. Inflamed plaques produce numerous proteases that cleave the ECM proteins in the fibrous cap and weaken the cap structure, and it is well established that inflammation-induced ECM proteolysis promotes plaque rupture. The major classes of proteinases expressed in atherosclerotic plaques are MMPs (MMP1, 2, 8, 9, 13, 14), and cathepsins (S, K, L) [33–39]
As plaques expand, inflammation promotes macrophage and SMC-mediated calcium deposition within the plaque and plaque calcification heightens plaque vulnerability by increasing stiffness and biomechanical inhomogeneity [40]. Plaque calcification occurs in response to cytokines that alter local expression of the proteins that promote calcium deposition (e.g., alkaline phosphatase (ALP), osteopontin, Runx2/Cbfa1, osteocalcein, members of the Notch family) and inhibit calcium resorption (e.g., Matrix Gla-protein (MGP), fetuin, osteoprotegerin). In clinical studies, vascular calcification markedly increases the risk of acute cardiovascular events and sudden cardiovascular death [41, 42].
1.4.2 Plaque Erosion
Plaque erosion, which accounts for ~20–25 % of fatal MIs, predominantly affects young women and patients with dyslipidemia [43]. Plaque erosion occurs when there is local desquamation of luminal endothelial cells in the absence of physical signs of fibrous cap rupture. In the setting of plaque erosion, exposure of the subendothelial ECM stimulates clotting cascade activation and arterial thrombosis. Unlike plaque rupture, cap thinning, and macrophage do not appear to be associated with plaque erosion. In fact, plaques undergoing erosion tend to have a high content of SMCs and proteoglycans [44]. Although the drastic differences in composition between rupture and erosion prone plaques suggest that the two processes have different pathologic mechanisms, the molecular mechanisms of plaque erosion are largely unknown. Notably, patients with plaque erosion have high levels of circulating myeloperoxidase (MPO), and in vitro analysis has demonstrated that MPO and its associated ROS (hypochlorous acid) can trigger EC apoptosis which promotes EC sloughing and erosion [45].
1.4.3 Intraplaque Hemorrhage (IPH)
IPH is an important contributor to plaque destabilization and in some cases can promote acute plaque rupture. The neovessels which develop in complex atheroma are fragile and prone to rupture. Following neoangiogenic rupture, the influx of immune cells, red blood cells (RBC), and blood proteins dramatically influence plaque biology.
ROS, oxidized LDLs, and lipids extracted from human atheromatous plaques promote RBC lysis and hemoglobin release [46, 47]. Within the plaque, free hemoglobin amplifies the oxidative stress responses which drive EC damage, apoptosis, and accumulation of necrotic debris [48, 49]. Blood-derived proteases also act on plaque ECM components and promote fibrous cap thinning and rupture. In line with this concept, studies have demonstrated that markers of IPH associate with rupture prone vulnerable plaque morphology [50]. Plaque neutrophil infiltration also increases following IPH, and the neutrophil-derived proteases including tissue plasminogen activator (t-PA), urokinase plasminogen activator (u-PA), plasmin, gelatinase, and serine proteases also degrade fibrous cap components and increase chance of rupture [51]. In addition to directly degrading ECM component of the fibrous cap, neutrophil-derived serine proteases break down atheroprotective SMC-secreted heat shock proteins (HSP) [52, 53].
1.5 Atherosclerosis Imaging
Advanced imaging strategies have a central role in the detection, treatment, and prevention of atherosclerotic vascular disease and its ischemic complications. The insidious nature of atherosclerosis is such that plaque development continues for years until lumen obstruction or thrombotic complications result in clinically apparent symptoms. The majority of acute CV events occur in patients who were previously asymptomatic, and thus, there has been a concerted effort to develop novel diagnostic imaging strategies that can identify patients before atherosclerotic complications occur. Once identified, the goal would be to treat these patients with risk-reduction therapies. In the following section, we will describe the general categories of CV imaging techniques with a focus on the vascular pathology which can be interrogated with each method. This general introduction will frame subsequent in-depth chapters which are focused on specific imaging modalities.
1.6 Anatomic Atherosclerotic Plaque Imaging
Several types of anatomical imaging methods are available to study cardiovascular anatomy and vascular structures. These modalities can be stratified by whether they involve noninvasive or invasive imaging methods (Table 1.1). With regard to vascular imaging of atherosclerosis, anatomic imaging provides excellent assessment of blood vessel structure and lumen stenosis; however, most anatomic techniques provide little information about plaque physiology or composition (as reviewed in depth [54]).
Table 1.1
Invasive and noninvasive imaging modalities
Technology | Resolution | Depth | Imaging moieties | |
---|---|---|---|---|
Noninvasive | ||||
CT/CTA | X-ray | 50 μm | No limit | Iodinated molecules |
MRI/MRA | Magnetic | 10–100 μm | No limit | Gd chelates, SPIO, USPIO |
PET/SPECT | Nuclear | ~2 mm | No limit | 18F, 64Cu, 11C Tracers/99mTc,123/124/125/131I,111In tracers |
Invasive | ||||
Angiography | X-ray | ~0.5–1 mm | No limit | Iodinated molecules |
OCT/OFDI | Optical | ~10 μm | 1–3 mm | None |
Angioscopy | Optical | 10–50 μm | Surface imaging | None |
IVUS/IVUS-VH | Ultrasound | ~150 μm | 4–10 mm | None |
1.6.1 X-Ray Computed Tomography
X-ray computed tomography (CT) is a noninvasive method that uses high-resolution X-ray imaging systems to generate images of cardiac and vascular structures. CT angiography (CTA) involves the intravenous administration of iodinated contrast agents to visualize luminal features of blood vessels (Fig. 1.3). Although CTA is a rapid and noninvasive method for assessment of flow-limiting peripheral and coronary artery stenosis, the spatial resolution of CTA is less than invasive coronary angiography (see below). Thus, invasive coronary angiography has superior sensitivity for identifying obstructive stenosis in medium- and small-caliber blood vessels. CTA also performs poorly in calcified or previously stented arteries where imaging artifacts limit interpretation of lumen patency. When compared to invasive angiography, the sensitivity and specificity of CTA for detecting flow-limiting stenosis of 50 % or greater are typically 96 and 86 %, respectively [55].
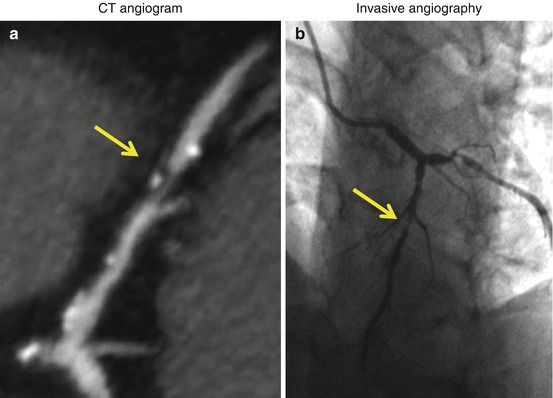
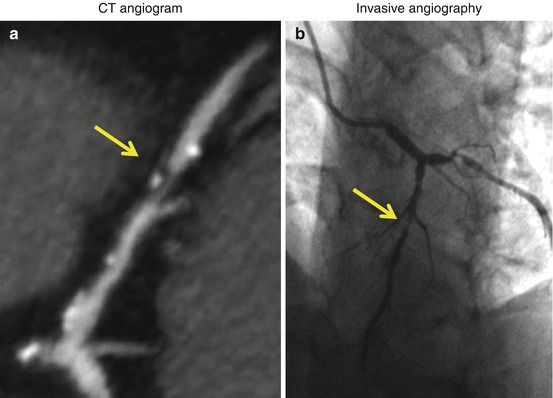
Fig. 1.3
Computed tomography coronary angiogram (a) and invasive coronary angiogram (b) in the same patient. Yellow arrows denote an obstructive atherosclerotic plaque in the mid-segment of the left anterior descending artery (LAD)
1.6.2 Magnetic Resonance Imaging
Magnetic resonance imaging (MRI) is another noninvasive cardiovascular imaging method that utilizes a magnetic field and radiofrequency detection to visualize cardiovascular structures with high spatial and temporal resolution. MRI frequently utilizes intravenous gadolinium-contrast enhancement to perform magnetic resonance angiography (MRA) (Fig. 1.4). Although MRA does an excellent job imaging large arteries and veins, its clinical use for the assessment of flow-limiting coronary artery stenosis has been hampered by significant limitations in visualizing distal segment and small branch disease. With the advent of advanced 3 tesla MRI systems, there is renewed interested in developing MRA for diagnostic imaging of obstructive coronary artery disease [56–58]. In research applications, combination of cardiovascular MRI with an invasive intracoronary MRI detector coil enables characterization of lipid-rich, fibrotic, and calcified plaques with favorable sensitivity and specificity [59].
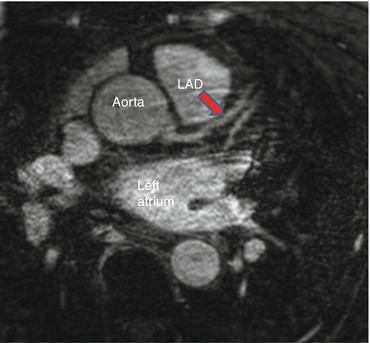
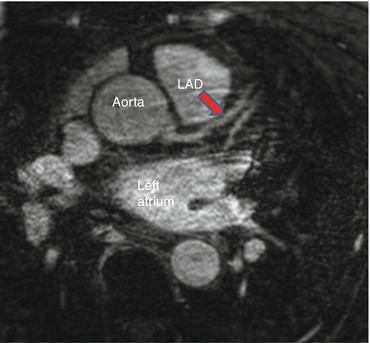
Fig. 1.4
Magnetic resonance angiogram (MRA) showing origin of the left main coronary artery from the aorta, giving rise to the left anterior descending artery (LAD), Red arrow
1.6.3 Angiography
Angiography is an invasive cardiovascular imaging technique that involves direct injection of an iodinated radiopaque contrast agent into the blood vessel to enable imaging with an X-ray-based video fluoroscopy technique (Fig. 1.5). Historically, angiography has been the gold standard for diagnosing obstructive vascular disease because it has excellent sensitivity and specificity and is frequently coupled with invasive methods to treat obstructive vascular disease with angioplasty and stenting. Although angiography is excellent at outlining the luminal border of the vasculature, this method provides no information about blood vessel structure or size beneath the endothelial surface.
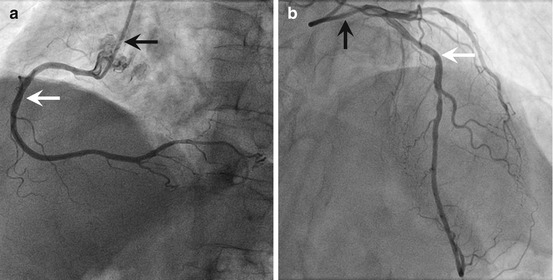
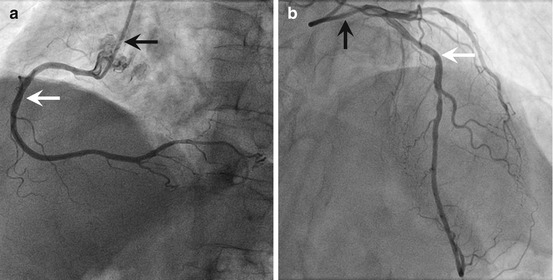
Fig. 1.5
Coronary angiogram. (a) Angiogram of a right human coronary artery. (b) Angiogram of a left human coronary artery prominently showing the left anterior descending artery (black arrow denotes the injection catheter; white arrow denotes the artery)
1.6.4 Angioscopy
Angioscopy is an invasive imaging modality that utilizes a high-intensity light source and optical imaging fibers to produce a full-color, 3-dimensional picture of the artery surface. Angioscopy requires that the imaging catheter be placed into the lumen of the artery, and because angioscopy utilizes standard light-based imaging, image acquisition cannot be done in the opaque blood medium. Thus, the angioscopy method requires occlusion of the artery with a balloon that enables arterial perfusion with a clear medium such as saline during the imaging sequence. Angioscopy generates images of the coronary artery lumen; however, it is only able to focus on a small part of the artery at a single time (Fig. 1.6). The limited imaging area and cumbersome process of balloon occlusion and saline perfusion have limited the widespread use of angioscopy in clinical practice or for research applications.
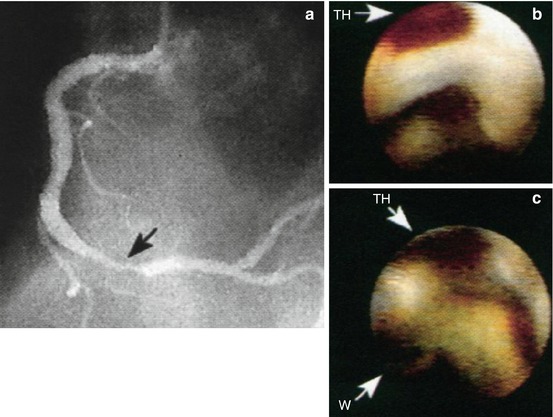
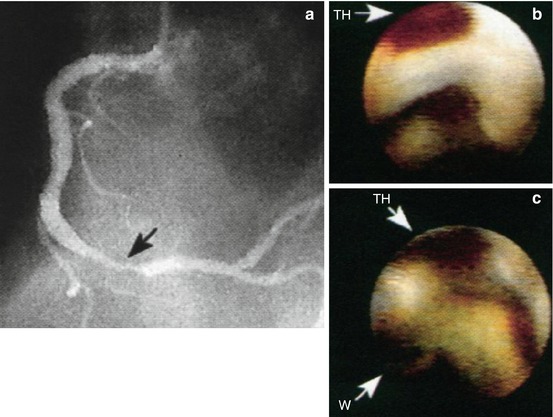
Fig. 1.6
Coronary artery angioscopy. (a) Angiogram of the right coronary lesion (Black arrow) in a patient with unstable angina. (b) Before percutaneous angioplasty, angioscopy shows mural thrombus (TH), (White arrow). (c) After percutaneous angioplasty, angioscopy shows the guidewire (W) in the lumen and persistent mural thrombus (TH) within an intimal dissection (White arrows) (Adapted from White et al. [187])
1.6.5 Intravascular Ultrasound
Intravascular ultrasound (IVUS) is an invasive vascular imaging method that utilizes an intracoronary ultrasound catheter that is placed into the lumen of the artery. The IVUS catheter contains a small ultrasound probe which employs reflected sound waves to generate an image of the blood vessel structure (Fig. 1.7). Standard clinical IVUS catheters have a spatial resolution of 150–200 μm and can image 4–10 mm deep into the blood vessel wall. IVUS can operate without balloon occlusion because the presence of blood does not impede sound wave imaging. IVUS imaging enables analysis of lumen and vessel dimensions and the distribution of atherosclerotic plaques. Because of the high spatial resolution, IVUS provides information about intravascular anatomy that far exceeds the level of detail obtained from conventional contrast angiography.
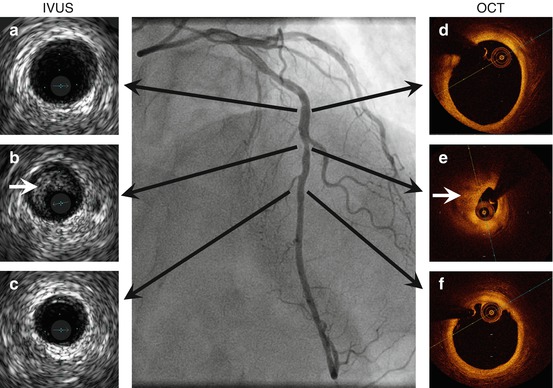
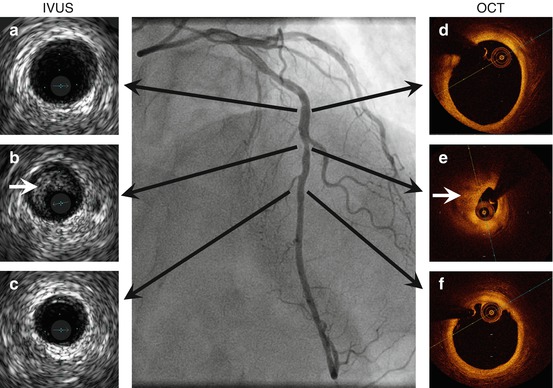
Fig. 1.7
Intravascular ultrasound and optical coherence tomography of the left anterior descending coronary artery. IVUS (left) and OCT (right). Black arrows denote corresponding cross section of the artery coregistered to the angiogram. Panels (B) and (E) show a stenotic atherosclerotic plaque (white arrow). Panel A-C, LAD IVUS images. Panel D-F LAD OCT images.
A recent adaptation of IVUS imaging has been termed virtual histology IVUS (VH-IVUS). VH-IVUS utilizes radiofrequency of reflected ultrasound signals and frequency domain analysis to generate a color-coded tissue map of plaque composition which is superimposed on the standard gray scale IVUS cross-sectional images. VH-IVUS improves characterization of the lipid content and necrotic core of atheroma, and in small studies, VH-IVUS-correlated plaque lipid content increased risk of adverse embolization events during therapeutic coronary artery intervention [60].
1.6.6 Optical Coherence Tomography
Optical coherence tomography (OCT) is an invasive intravascular imaging modality that utilizes near-infrared light to generate cross-sectional blood vessel images. OCT catheters, placed into the blood vessel lumen to enable imaging, generate images of coronary arteries with 10–15 μm of spatial resolution that is an order of magnitude more precise than the 150–200 μm resolution of IVUS (Fig. 1.7). Although the spatial resolution of OCT is markedly superior to IVUS, near-infrared light does not penetrate tissue as effectively as sound, and thus, OCT can only image from 1 to 3 mm into the vessel wall. In addition, because near-infrared light is scattered by red blood cells, OCT is similar to angioscopy because it requires transient blood clearance during image acquisition. Importantly, recent technological advances in OCT console and catheter design (i.e., optical frequency domain imaging (OFDI)) have enabled rapid imaging element pullback that allows image acquisition to occur during the brief blood-free interval when standard angiography contrast is injected into the artery. This improvement in catheter speed eliminated the need for the arterial balloon occlusion, and OCT imaging is now a convenient, high-resolution imaging modality that is widely used in many centers. In addition, several recent studies have demonstrated that OCT is a highly effective method for identifying and quantifying thin cap fibroatheroma which are predisposed to plaque rupture [61–63].
1.7 Functional Atherosclerotic Plaque Imaging
Although anatomic imaging methods provide some insights into plaque lipid composition, fibrous cap thickness, and plaque volume, anatomic imaging methods lack the ability to define critical components of plaque physiology that predict plaque complications. These physiological characteristics such as plaque inflammation, metabolic activity, and protease function are key determinants of atheroprogression and clinical complications of atherosclerotic disease. In the past decade, we have made great strides in our understanding of the molecular processes that promote plaque progression, vulnerability, and atherothrombotic events. Plaque characterization with molecular phenotyping can now be done using a spectrum of imaging modalities. Advances in molecular imaging methods greatly facilitate research on vascular disease mechanisms, and several molecular and functional imaging techniques have the potential to be used in the clinical setting to identify vulnerable plaques, to follow disease activity, and to monitor therapeutic responses. In general, functional imaging methods employ specific molecular imaging probes that are linked to one or more detectable imaging constituents such as fluorophores, radionuclide compounds, magnetic particles, or microbubbles.
The detection methods for functional imaging vary considerably. Nuclear, positron emission tomography (PET), and single-photon emission computed tomography (SPECT) rely on radioactive tracers for imaging detection, and these method often have high sensitivity and quantitative ability but relatively low spatial resolution. Optical modalities for functional imaging typically utilize fluorescent detectors and probes that are in the near-infrared (NIR) spectrum to visualize vascular tissues. Fluorescent detection methods include confocal and multiphoton microscopy for invasive intravital imaging and fluorescent tomography for noninvasive imaging. The limited penetration of light through tissues currently prevents the noninvasive use of optical and fluorescent methods to image the human vasculature. The invasive, catheter-based, InfraRedx Lipiscan™ combines IVUS and intravascular fluorescence to define vessel architecture and plaque lipid content [64]. The following sections will highlight physiologic processes and specific targets that are the focus of current functional and molecular imaging techniques (Table 1.2, Fig. 1.8).
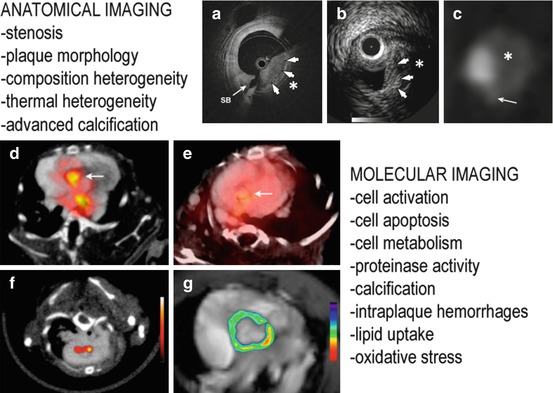
Table 1.2
Molecular targets of atherosclerotic plaques and associated imaging moieties and modalities
Biological process | Molecular target | Imaging moieties | Imaging platforms |
---|---|---|---|
Adhesion | VCAM-1 | 123I, 99mTc, 18F, SPIO, NIRF, microbubbles | PET/SPECT, MRI, optical imaging, CEU |
ICAM-1 | Gd, microbubbles | MRI, CEU | |
E-selectin | SPIO | MRI | |
P-selectin | SPIO, Gd, microbubbles | MRI, CEU | |
Phagocytic activity | – | SPIO | MRI |
– | 64Cu, 18F-SPIO | PET/SPECT | |
Metabolic activity | FDG | 18F | PET |
FCH | 18F | PET | |
Lipid uptake | HDL/LDL | 123I, 125I, 131I, 111In, 99mTc, Gd | PET/SPECT, MRI |
CD68 | 124I | PET/SPECT | |
LOX-1 | 111In, 99mTc, Gd | SPECT, MRI | |
SRs | Microbubbles | CEU | |
Oxidative stress | Oxidized epitopes | 125I, Gd, SPIO | PET/SPECT, MRI |
MPO | Gd | MRI | |
Cell death | Phosphatidylserine | 123I, 124I, 99mTc, Gd, SPIO | PET/SPECT, MRI |
Proteinase activity | MMPs | 123I, 99mTc, 18F, Gd, SPIO, NIRF | PET/SPECT, MRI, optical imaging |
Cathepsins | NIRF | Optical imaging | |
Collagen | Gd, NIRF | MRI, optical imaging | |
Neoangiogenesis | αvβ3 integrin | 18F, Gd, NIRF, microbubbles | PET, MRI, optical imaging, CEU |
– | MR-contrast agent (Ktrans), microbubbles | MRI, CEU | |
Osteogenesis | Ca++ hydroxyapatite | 18F, NIRF | PET, optical imaging |
Thrombosis | Glycoprotein IIb/IIIa | 99mTc, SPIO, NIRF, microbubbles | PET, MRI, optical imaging, CEU |
Fibrin | 64Cu, Gd | PET, MRI | |
Factor XIII | Gd, SPIO, NIRF | MRI, optical imaging |
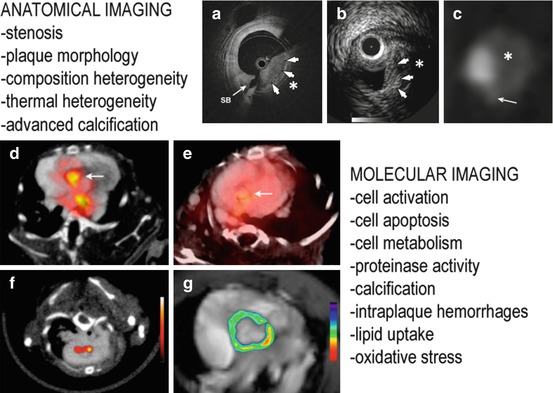
Fig. 1.8
Anatomical and molecular imaging for atherosclerosis. Top panels: OCT optical frequency domain imaging (OFDI) (a) shows a thick fibrous cap (white arrows) and a large necrotic core with typical low-intensity appearance. IVUS (b) demonstrates a narrowed coronary lumen with a focal heterogeneous plaque overlapped by a fibrous layer (white arrows). The signal drops out due to the plaque thickness. Computed tomography angiography (c) detects a large noncalcified plaque segment. Bottom panels: (d) VCAM-1 expression in atherosclerosis using 18F −4 V PET/CT. (e) Lower uptake is seen in ApoE−/− mice treated with statins. (f) PET/CT with macrophage targeting nanoparticles. (g) MRI with pseudocolored T2-weighted signal intensity, which decreased because of accumulation of iron oxide nanoparticles (Adapted from Refs. [14, 15, 188, 189])
1.7.1 Cell Activation Imaging
Endothelial and inflammatory cell activation is an attractive target for functional imaging of atherosclerosis because vascular inflammation (1) is increased in early atherogenesis, (2) correlates with atheroprogression, and (3) is a marker of plaque vulnerability. VCAM-1 is an adhesion molecule expressed by activated ECs and macrophages at sites of vascular inflammation and within inflamed atherosclerotic lesions. Various imaging strategies have targeted VCAM-1 expression with very late antigen-4 (VLA-4) peptides, anti-VCAM-1 antibodies, or an MHCI peptide that interacts with VCAM-1. These VCAM-1 targeting moieties have been coupled to radionuclides (123I, 99mTc, 18F), near-infrared fluorescent dyes, superparamagnetic iron oxide (SPIO) particles, or microbubbles. After injection, these probes accumulate in inflamed tissues and on activated cells, with little or no toxicity [65–71]. Preclinical analysis in mouse models established that there is selective increase in VCAM-1 probe concentration in atherosclerotic lesions in vivo and that probe uptake correlates with lipid content and VCAM-1 expression [72–76].
Similar strategies target other cell adhesion molecules that play a critical role in the pathobiology of atherosclerosis. Specific probes for ICAM-1, E-selectin, and P-selectin effectively identify activated endothelial cells in atherosclerotic lesions [77–81]. Theranostic imaging strategies have coupled molecular imaging with therapeutic compound delivery; ICAM-1 antibody-targeted echogenic immunoliposomes that contain NO donors deliver vasculoprotective NO to areas of inflamed EC [82]. Combination of targeting moieties for adhesion molecules increases the sensitivity and specificity of inflammation imaging and can provide information about the colocalization of physiologic processes and anatomic structures in vivo [67, 83–85].
1.7.2 Phagocytosis Imaging
Macrophages are the predominant immune cells in atherosclerotic plaques and their presence correlates with plaque inflammation and risk of rupture [86]. To detect macrophage accumulation in lesions, researchers developed iron-based MRI probes (i.e., superparamagnetic iron oxide probes (SPIO, >50 nm) and ultrasmall superparamagnetic iron oxide (USPIO, <50 nm) probes) that are engulfed and concentrated in plaque-resident macrophages. These iron MRI compounds were first in class among a growing number of novel enhanced MRI nanoagents. The prototypical phagocytosis MRI agent has an iron oxide crystal core and a synthetic monomeric or polymeric polysaccharide coating [87, 88]. Phagocytic cells avidly take up these nanoparticles which causes them to accumulate preferentially in inflamed plaques that have large number of activated macrophages [89–91]. Macrophage phagocytic probes have been used to assess the effect of atherosclerosis therapies. In the ATHEROMA study (Atorvastatin Therapy: Effects on Reduction of Macrophage Activity), atorvastatin reduced plaque USPIO uptake in humans which is reflective of decreases in macrophage-mediated plaque inflammation [92]. The plaque specificity of agents that target macrophage phagocytic activity can be increased by conjugating macrophage-avid nanoparticles with lipoproteins that undergo receptor-mediated endocytosis or by conjugating particles with phosphatidylserine (PS)-coated vesicles that mimic apoptotic bodies (discussed in detail below) [93, 94].
In experimental models of atherosclerosis, dual coupling of radiolabeled elements (64Cu, 18F) and/or fluorescent dyes to nanoparticles allows combined multimodal optical and PET/SPECT imaging of plaque [95, 96]. Combination imaging strategy offers the benefits of high spatial anatomic resolution of fluorescence and excellent sensitivity and quantification of PET/SPECT. CT imaging also has the potential to detect plaque-resident macrophages. In experimental rabbit studies, high-resolution CT readily detects iodinated nanoparticles within inflamed macrophage-rich plaques [97].
1.7.3 Imaging of Lipid Uptake/Foam Cell Formation
Macrophage lipid uptake is critical to the pathobiology of early plaque development when foam cell formation is occurring, and heightened lipid uptake also occurs in lipid-rich plaques that are vulnerable to plaque rupture [98]. Lipid-based imaging agents can potentially identify areas of early plaque formation and progression. These probes include high-density lipoprotein (HDL) and LDL compounds conjugated to radioisotopes for nuclear imaging (123I, 125I, 131I, 111In, 99mTc) or magnetic compounds for MRI visualization [99, 100]. As discussed above, monocytes and macrophages engulf lipids using specific scavenger receptors, and thus, an alternative approach for imaging lipid uptake involves selective targeting of lipoprotein receptors such as LOX-1 or CD68. A preclinical study using 99mTc-labeled anti-LOX-1 antibody quantified rabbit atherosclerotic plaques by PET. In this study, signal enhancement colocalized strongly with postmortem LOX-1 plaque expression, albeit the signal intensity did not correlate with histological plaque grading [101]. LOX-1 targeted USPIO compounds are promising MRI agents that colocalize with plaque macrophage accumulation [102]. LOX-1 targeting moieties also function well in MR, PET, and fluorescent imaging investigations [103]. NIRF-conjugated anti-oxLDL antibodies enable imaging of plaque progression and statin-induced plaque regression by fluorescent reflectance imaging in mice [104].
1.7.4 Imaging Metabolic Activity
Plaque progression strongly correlates with vascular cell proliferative activity and with heightened metabolic activity of cells within the atheroma. Several studies demonstrate that atherosclerotic plaques have greater metabolic activity compared to healthy vascular tissue, and this increased metabolic state can be exploited to study plaque physiology [105]. Metabolically active cells readily uptake glucose and its analog, fluorine-labeled 2-deoxy-D-glucose (FDG). 18F-FDG is widely used to image the myocardium by PET, and it is currently under development as a vascular imaging agent. Early work demonstrated that FDG uptake in plaques colocalizes with macrophages more than with SMCs [106, 107]. Furthermore, in vivo preclinical studies provide evidence that 18F-FDG PET signal is increased in atherosclerotic rabbit aortas compared to controls [107]. Recently, experimental work using human tissues confirmed that FDG selectively accumulates in inflamed atheromatous plaques [108–116], and clinical studies have showed that FDG PET can be used to follow beneficial vascular effects of lipid or lifestyle therapies aimed at reducing atherosclerotic risk [117–125].
In order to enhance the signal to noise ratio of cardiovascular metabolism imaging, scientists are developing new metabolic biotracers that will preferentially enter plaque and/or macrophages over the metabolically active myocardium. Indeed, compared to the myocardium, macrophages have a higher avidity for the PET agent 18F- fluorocholine (FCH), which incorporates into the cell membrane. In preclinical studies, macrophages in mouse atherosclerotic lesions take up FCH more readily than FDG [126]. Further investigations are needed to determine if FCH performs better than FDG for human plaque imaging.
Intravascular thermography (IV thermography) is an invasive method that examines plaque metabolic activity. Thermography is similar to angioscopy, IVUS, and OCT in that it requires placement of a catheter into the artery. The thermography catheter utilizes a miniaturized thermal sensor to measure temperature heterogeneity along the length of the blood vessel. Heightened plaque inflammation and metabolic activity are associated with increase cellular thermogenesis, and thus, inflamed or proliferative plaques can be identified because they have an increased temperature and greater thermal signals. Although elevated IV thermography temperature signals have been shown to correlate with plaque inflammation [127], this technology is still under development and is not currently approved for widespread clinical use.
1.7.5 Apoptosis Imaging
Cellular apoptosis contributes prominently to accumulation of the debris that comprise the necrotic core of advanced atheroma. In addition, plaque inflammation is associated with increased apoptosis because several inflammatory cytokines activate apoptotic pathways in vascular cells [128]. In non-apoptotic cells, there is asymmetric phospholipid distribution of the cellular membrane in which negatively charged PS is sequestered on the cytosolic (inner) layer of bilipid membrane. Upon activation of apoptotic pathways, PS becomes present on the outer surface of the cell, and thus, PS is a marker of cellular apoptosis and PS-targeted imaging agents can enable visualization of cell death.
One of the most widely used PS probes is the protein annexin V, which binds avidly to PS-containing cell membranes. Iron and radiolabeled annexin V (123I, 124I, 99mTc and 18F) identify plaques, plaque macrophages, and vascular apoptosis by MRI and SPECT [129–133]. Notably, 99mTc-annexin V concentrates preferentially in lesions that contain macrophages and necrotic debris compared to lesions that have a non-inflamed fibrous core [132]. In a clinical study of orthotopic heart transplant patients who were suffering from rejection, there was increased uptake of 99mTc-annexin radiotracer in areas of myocardial infarction [134]. Based on early success in preclinical studies, new investigations are underway to examine whether annexin V probes can quantify the vasculoprotective anti-apoptotic effects of angiotensin inhibitory medications [135].124I-hypericin is a new necrosis-avid PET agent that accumulates in areas of cell death. Preliminary studies using 124I-hypericin demonstrate enhancement of carotid plaques in atherosclerotic mice [136, 137].
1.7.6 Oxidative Stress Imaging
Oxidative stress markedly increases in atherogenic conditions, and ROS promote inflammatory cell activation, lipid oxidation, and apoptosis events that drive plaque development and progression [138]. One method of oxidative stress imaging has focused on developing antibodies that detect by-products of lipid oxidation. Studies that employed radionuclide or MRI-conjugated antibodies against oxidized malondialdehyde-lysine (MDA) or oxidized phospholipid epitopes (e.g., MDA2, E06, and IK17) showed the feasibility of assessing qualitatively and quantitatively oxidative stress in atherosclerotic lesions [139, 140]. Another strategy for oxidative stress imaging involves targeting the ROS-producing enzymes that are made by cells within atherosclerotic plaques. MPO is an enzyme that generates hypochlorous acid (HOCl/OCl-) which is a marker of oxidative stress. To detect MPO activity, investigators designed a small-molecule, gadolinium-based MRI sensor for MPO activity. This MPO probe detected increased MPO activity in the infarcted myocardium of mice 2 days after coronary artery ligation. Moreover, serial imaging sessions with this MPO agent accurately reflected the therapeutic beneficial effect of statins after ischemia-reperfusion injury [141]. Similarly designed fluorescent MPO nanosensors have also been used to detect ROS in preclinical studies [142].
1.7.7 Angiogenesis Imaging
The utility of neoangiogenesis imaging extends well beyond the realm of cardiovascular disease, and much of the pioneering work in this field has been applied to the study of tumor angiogenesis in oncology. Many angiogenic imaging agents target expression of proteins that are critically involved in new blood vessel growth. The cell surface receptor ανβ3 is an integrin adhesion molecule that regulates cell migration, proliferation, and survival [143]. ανβ3 expression increases during angiogenesis, and Arg-Gly-Asp (RGD) peptides that bind to this integrin are effective angiogenesis probes. Ferromagnetic RGD MRI probes enable detection of angiogenesis in the abdominal aorta of atherosclerotic rabbits and rats [144, 145]. These imaging agents also effectively quantify reduction in angiogenesis following treatment with antiangiogenic drugs [146, 147]. Several investigations have also utilized PET-, optical-, and CEU-based ανβ3 integrin probes to assess plaque burden in animal models of atherosclerosis [148–150]. With regard to atherosclerosis imaging, it is currently unclear whether ανβ3 imaging techniques are detecting neoangiogenic process or the accumulation of macrophages in lesions, because macrophages and angiogenic cells both express ανβ3 receptors [148].
One method of angiogenesis imaging exploits the fact that atherosclerosis-associated angiogenesis results in the production of fragile blood vessels that have markedly increased vascular permeability. Because neoangiogenic vessels have increased permeability, intravascular contrast agents can detect the presence of new vessels based on the leakage of intravascular contents into the perivascular space. Dynamic contrast-enhanced MRI is an angiogenesis imaging method that detects the extravascular presence of an MRI contrast agent. This functional imaging method relies on altered blood vessel physiology rather than a singular molecular target to obtain information about angiogenic responses.
1.7.8 Proteinase Imaging
Proteinases are important catalytic enzymes that play a central role in all aspects of atherosclerotic plaque biology. These enzymes, which include matrix metalloproteinases (MMP) and cathepsins, directly break down interstitial fibrillar collagen and elastin and contribute to fibrous cap thinning and plaque rupture. Imaging “smart” probes for proteinases take advantage of their proteolytic activity because the probe signal intensity increases dramatically upon cleavage by the proteinase.
Investigators have successfully imaged vascular MMP activity in preclinical models using a NIRF MMP-cleaved smart probe [151, 152]. Pharmacotherapy experiments demonstrated that NIRF MMP smart probes can quantify the therapeutic effect of MMP-13 and peroxisome proliferator-activated receptor PPARγ inhibitors on plaque MMP activity and macrophage accumulation [153, 154]. NIRF smart probe technology has been adapted to image the activity of several plaque proteinases including MMP-2 and MMP-9 [155], and cathepsin B, C, and S [38, 156–158]. The majority of these NIRF smart probes have only been tested in animal models, and there are technical hurdles related to the fact that most smart probes have poor plaque penetration and rapidly diffuse out of the plaque once they are activated. To address the issue of probe diffusion and the overall low sensitivity of NIRF smart probes agents, investigators have developed invasive intraluminal optical imaging catheters that can detect NIRF probes with higher sensitivity and spatial resolution than noninvasive detectors [159]. New activatable, cell-penetrating NIRF peptide probes may also improve NIRF proteinase imaging because these agents are retained and concentrate within cells following proteinase cleavage [160, 161].
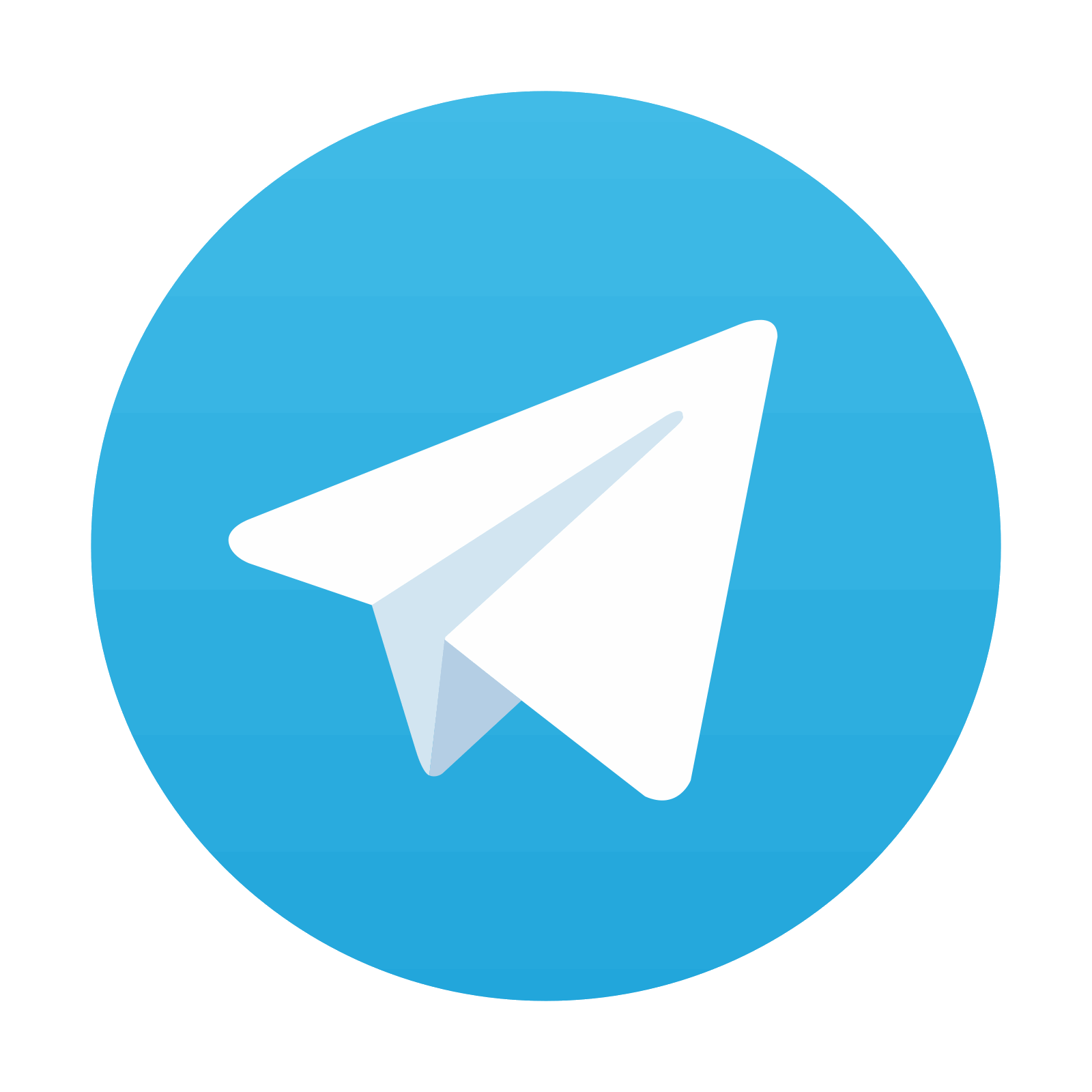
Stay updated, free articles. Join our Telegram channel
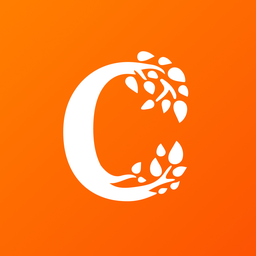
Full access? Get Clinical Tree
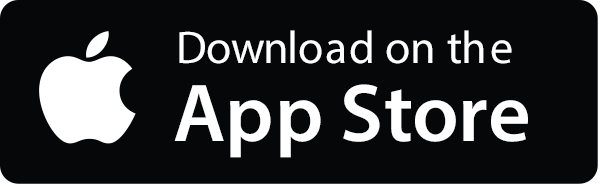
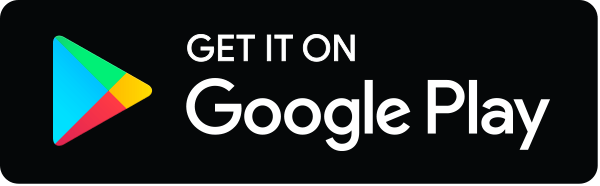