and Ashutosh Dash2
(1)
Nuclear Security and Isotope Division, Oak Ridge National Laboratory, OAK RIDGE, USA
(2)
Isotope Production and Applications Division, Bhabha Atomic Research Centre, Mumbai, India
10.1 Introduction
Peptides are usually classified as containing less than 50 amino acid residues (~5500 Da) and consist of any combination of amino acids linked by peptide bonds. Many tumors overexpress specific cell-surface receptors which allow the development of therapeutic peptides which can target these cells for treatment of a wide variety of cancers. Among the different peptides used for tumor targeting, somatostatin analogs (SST) have attracted unprecedented attention as a treatment modality for patients with inoperable or metastasized endocrine gastroenteropancreatic (GEP) tumors (Okarvi 2004; Heppeler et al. 2000; Van Den Bossche and Van de Wiele 2004), discussed later in this chapter in Sect. 10.3.2. The breadth of research on targeted peptides is very broad and has, in particular, evolved into a key clinical specialty in nuclear medicine and oncology for targeted tumor therapy. Table 10.1 illustrates examples of a variety of radiolabeled peptides which have been evaluated for cancer cell targeting, many of which have entered the clinical arena.
Table 10.1
Key examples of regulatory peptides and their receptors overexpressed on tumors
Peptide | Number of amino acid residues | Receptor types/subtypes | Tumor expression |
---|---|---|---|
α-MSH | 13 | α-MSH-R | Melanomas |
Bombesin/GRP | 14 | BB1 (NMB-R), BB2 (GRP-R), BB3, BB4 | Prostate, breast, pancreas, gastric, colorectal, small-cell lung cancer |
CCK/gastrin | 17 or 34 | CCK1, CCK2 | Medullary thyroid cancer, small-cell lung cancer, gastrointestinal stromal tumor, stromal ovarian cancer, astrocytomas |
LHRH | 10 | LHRH-R | Prostate, breast cancer |
Neurotensin | 13 | NTR1, NTR2, NTR3 | Small-cell lung cancer, colon, exocrine ductal pancreatic cancer, Ewing sarcoma, meningioma, astrocytoma, breast, prostate cancer |
RGD | αvβ3-integrin | Glioma, breast, prostate cancer | |
Somatostatin | 14 or 28 | SST1, SST2, SST3, SST4, SST5 | Neuroendocrine tumors lymphoma, paraganglioma, carcinoids, breast, brain, renal, small-cell lung cancer, medullary thyroid cancer |
Substance P | 11 | NK1, NK2, NK3 | Glial tumors (glioblastoma, medullary thyroid cancer), pancreas, breast, small-cell lung cancer |
VIP | 28 | VPAC1, VPAC2 | Adenocarcinomas of breast, prostate, stomach, and liver; neuroendocrine tumors |
10.2 Amino Acids, Peptides, and Proteins
10.2.1 Amino Acids
The role of amino acids (Fig. 10.1) in the evolution of biological diversity is well established, and these biomolecules play a pivotal role as building blocks for a vast array of molecular signaling, signal transduction, and recognition of transformation peptide and protein units. The ability of these molecules to perform as neurotransmitters offers a pool of precursors for neurotransmitters, for instance, exemplified by the adrenergic, dopaminergic, and serotonergic systems. When assembled into small peptides, amino acids can generate a variety of hormones, releasing factors, neurotransmitters, and neuromodulators. Larger constructs of amino acids comprise molecular recognition systems such as immunoglobulins and receptors. On the other hand, assembly into enzymes provides catalyzing nearly the entire spectrum of bioorganic reactions occurring in living systems.
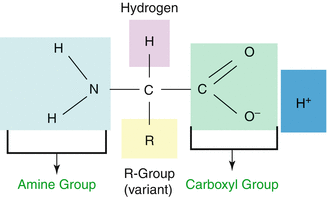
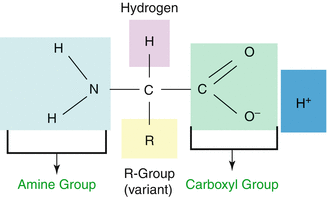
Fig. 10.1
Basic amino acid structure
10.2.2 Peptides and Proteins
Proteins are large macromolecules (Fig. 10.2) consisting of polymerized amino acid units connected by amide bonding and are essential to determine the structure as well as to perform most functions in living cells including structural components, enzyme catalysts, and other functions. There are about 20 different amino acids in nature which are assembled in chains of varying lengths to form proteins. The order of amino acids (primary structure) determines the structure and function of the protein. In contrast, peptides are much smaller molecules consisting of two or more amino acids linked together by amide (peptide) bonds in which the amino acid amine and carboxylic acid functional groups join together to form amide bonds of the peptide. Peptide thus consists of short- or medium-length-chain building blocks of amino acids. The size of peptides can roughly vary from molecules with only two amino acids to as many as 50.
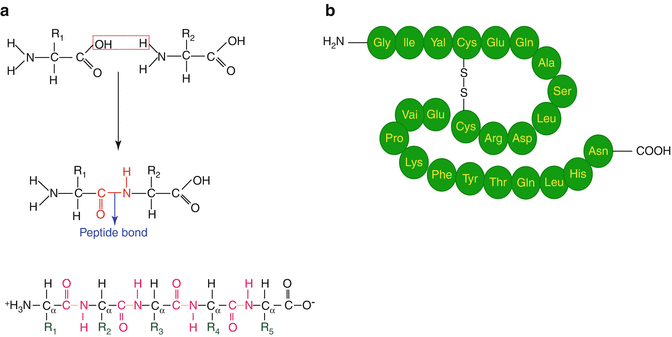
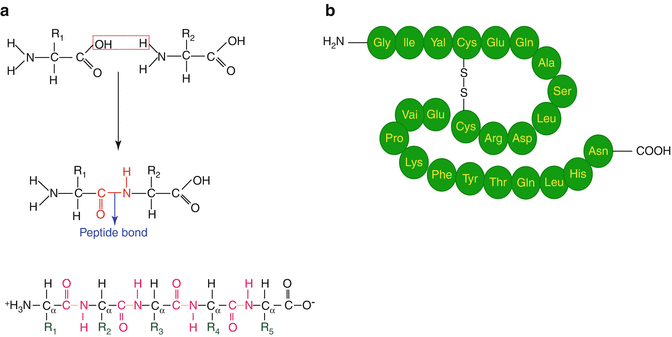
Fig. 10.2
(a) Condensation of amino acids to form the amide bond, and (b) primary polyamino protein structure illustrating disulfide bonding
The different amino acids and the order in which they are joined together by peptide bonds in a peptide or protein are referred to as the primary structure. In addition to dramatic differences in molecular size, proteins and peptides also differ in other ways, since proteins consist of only 20 different amino acids, while in peptides these 20 “protein” amino acids are found as well as other naturally available amino acids. Peptides are basically small proteins, having molecular weights less than 5,000 and do not possess a well-defined three-dimensional (tertiary) structure. On the other hand, proteins are typically much larger, with folded chains which have biological significance. As a result of this difference in size, proteins and peptides are also different in the types of three-dimensional structures and functionalities that they possess. In contrast to proteins, peptides generally do not possess a well-defined three-dimensional (tertiary) structure. Peptides not only exist in natural form but also can be prepared synthetically.
10.2.3 Regulatory Peptides
Peptides regulate most physiological processes, acting at some sites as endocrine or paracrine signals and at others as neurotransmitters or growth factors. Peptides that are primarily synthesized in the brain, especially in neurons, are called “neuropeptides” and function either directly or indirectly to modulate synaptic activity. In addition, “neuropeptides” may also function as primary neurotransmitters. Peptides are found and have important biological significance throughout the body, which includes the gut, lymphatic tissue, endocrine system, and many other tissues. The heterogeneous peptide hormones produced by the gastrointestinal tract control digestion and are secreted from cells that line the lumen of the gut, which include the mucosa as well as in endocrine organs such as the pancreas. Gut hormones also regulate cell growth, but in some instances, well-recognized peptides such as substance P and pancreatic polypeptide are recognized as gut hormones, but their function is still uncertain. However, these peptides can be secreted by endocrine tumor cells and may be responsible for some systemic manifestations. In general terms, regulatory peptides represent a group of different families of molecules known to act on multiple targets in the human body at extremely low concentrations.
Biologically active peptides are the products of genes, and their targets are proteins or protein-coupled receptors. These naturally occurring peptides play a regulatory role in the body and generally mediate their function through specific binding to receptors, such as the transmembrane G-protein-coupled receptors. Peptide-receptor binding to this protein can activate or inhibit biological processes via different mechanisms, including activation of G-proteins, tyrosine kinases, or transcription processes. Importantly for therapeutic radiopharmaceutical targeting, many of these receptors have been shown to be significantly overexpressed in certain diseases and especially in particular tumor types. The targeting of these overexpressed receptors in various tumors has thus generated tremendous interest in the development of specific peptides for diagnosis as well as therapy. A number of tumor receptors such as somatostatin (SST), integrin, gastrin-releasing peptide (GRP), cholecystokinin (CCK), R-melanocyte-stimulating hormone (R-MSH), and glucagon-like peptide-1 (GLP-1) receptors have been identified, and application of radioactive specific regulatory peptides targeted (Table 10.2) to these receptors for tumor receptor therapy is in various stages of development.
Table 10.2
Examples of key regulatory peptides in PRRT
Abbreviation | Name | Biological effects |
---|---|---|
α-MSH | α-Melanocyte-stimulating hormone | Melanogenesis |
ANF | Atrial natriuretic factor | Natriuresis, vasodilation |
AT | Angiotensin | Vasoconstriction |
BN | Bombesin/gastrin-releasing peptide | Gut hormone release |
BK | Bradykinin | Vasodilation, hypotension |
CCK | Cholecystokinin | Gallbladder contraction, exocrine pancreatic secretion |
CT | Calcitonin | Calcium homeostasis |
GAL | Galanin | Gastrointestinal immotility |
LHRH | Luteinizing hormone-releasing hormone | LH and FSH stimulation |
NPY | Neuropeptide Y | Induction of food intake, inhibition of anxiety |
NT | Neurotensin | Vasoconstriction, raise in vascular permeability |
SEC | Secretin | Pancreatic NaHCO3 secretion |
SST | Somatostatin | Inhibition of hormone and exocrine secretion |
SP | Substance P | Hypotension, salivary secretion |
VP | Vasopressin | Vasoconstriction, antidiuresis |
VIP | Vasoactive intestinal peptide | Vasodilation, water and electrolyte secretion in the gut |
10.2.4 Peptides as Therapeutic Vectors
The ability to target specific receptors expressed on the surfaces of human cancer cells offers the scope of using radiolabeled peptides as transport vehicles to guide the radionuclides to the tissues expressing a particular receptor (Rufini et al. 2006). These receptors constitute useful molecular targets for the treatment of cancer owing to their location on the plasma membrane. Due to their low molecular weight, they show rapid diffusion into target tissue, and upon binding of the radioligand, the receptor–ligand complex is often internalized, allowing long retention of radioactivity in tumor cells. They also generally exhibit rapid vascular clearance from nontarget tissues, resulting in high tumor-to-background tissue ratios, which is a goal to minimize radioactive dose to nontarget tissues. In addition, peptides generally are non-immunogenic, so do not illicit any unwanted response. The most dramatic example of successfully targeting of cellular receptors with radiolabeled peptides (Fig. 10.3) is represented by somatostatin receptors, which are overexpressed in a majority of neuroendocrine tumors such as gastrointestinal and bronchial neuroendocrine tumors, pituitary adenomas, paragangliomas, pheochromocytomas, and neuroblastomas. Several key important regulatory peptides along with their receptors which are overexpressed on tumor cells are summarized in Table 10.1 (Okarvi 2004; Reubi et al. 2005). Since most of these peptides act through multiple peptide receptor subtypes, it is crucial that the peptide receptor subtype expressed by a given tumor corresponds to the subtype to which the radioligand used for PRRT binds with high affinity.
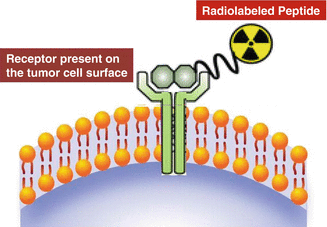
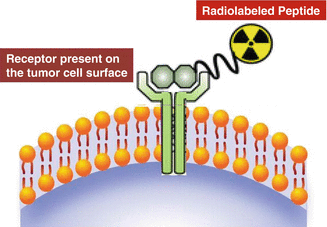
Fig. 10.3
Idealized illustration of radiolabeled peptide binding to a cell-surface receptor
10.2.5 Advantages of Peptides for Therapy
There are a variety of attractive properties for targeting small radiopeptides over other biologically active molecules for therapeutic applications (Reubi et al. 2005). The small size and low molecular weight of peptides facilitate rapid access to target tissue. Peptides are also easy to chemically synthesize using either an automated peptide synthesizer or by manual synthesis. Automated synthesis is used for rapid production of simple peptides, whereas manual synthesis is often more practical for time-consuming or difficult sequences and peptide modifications. High-specific-activity peptides can be prepared and used to minimize unwanted physiologic effects (i.e., eliciting physiological effects), and known sequences of amino acids can be modified to decrease their in vivo catabolic rate. Peptides also allow the possibility for radiolabeling with a variety of radionuclides by using both conventional and novel chelating moieties and also offer the feasibility of “kit” formulation as a practical strategy for the preparation of therapeutic agents. In addition, peptides are amenable to extensive chemical/molecular modifications to optimize their affinity for a particular receptor and to display a more specific biodistribution pattern. From a synthetic perspective, peptides have the ability to tolerate harsh conditions (pH, temperature, etc.) of chemical modifications and also the possibility for attachment of a chelating agent at the C- or N-terminus, as well to the free amine or carboxylic acid present on the peptide. Biologically, they have high affinity and specificity toward a wide range of target molecules. The specificity of the peptides toward G-protein-coupled receptors, which are overexpressed by cancer cells, identifies such peptides as ideal vectors for transportation of conjugated radionuclides to primary tumors and metastatic sites. Their small size and low molecular weight facilitate rapid penetration of peptides to target tissues, compared to the much larger proteins and antibodies. Another advantage usually displayed by peptides is the favorable pharmacokinetics characterized by high concentration in the target tissues and rapid clearance from the blood pool and nontarget tissues. In this context, elimination processes can be modified to accommodate a variety of possible routes of excretion or metabolism.
10.2.6 Limitations of Peptides for Therapy
Criteria for successful use of peptide tracers include high target specificity, high binding affinity, prolonged metabolic stability, and high target-to-nontarget background ratio. A short vascular half-life is a major road impediment for successful in vivo application of radiolabeled peptides because of susceptibility to degradation before reaching the intended target site. In order to circumvent such enzymatic destruction, most peptides can be synthetically modified. Considerable research efforts have been directed toward the development of metabolically stable peptides suitable for clinical use by the introduction of appropriate molecular modifications, such as the use of more stable D-amino acids instead of the naturally occurring L-amino acid isomer and the use of pseudopeptide bonds. In addition, the inclusion of amino alcohols and the insertion of unnatural amino acids or amino acid residues with modified side chains without compromising the receptor-binding affinity and biological activity of the peptide have been exceptionally successful (Okarvi 2004). Because of the ability of bioactive peptides to induce pharmacologic effects even in trace amounts, the capability of preparing high-specific-activity (radioactivity per mass) peptides is an important requirement. Another strategy which has been successfully implemented is the use of antagonists (receptor binding but no physiological response) rather than agonist (receptor binding eliciting physiological response) peptide entities in order to diminish unwanted physiological effects. The loss of receptor-binding affinity and in vivo peptide metabolism resulting from coupling with a chelator and/or introduction of a radiolabel is another major challenge which can be associated with the use of small peptides. This issue can be effectively circumvented by site-directed radiolabeling that can be achieved by inserting a spacer group between the binding sequence and the chelating moiety (Okarvi 2004). One successful appealing approach is the cyclization of the peptide around a metal core which not only makes the peptide analogs resistant to chemical and proteolytic degradation in vivo but also greatly increases the target receptor affinity (Chen et al. 1999; Miao et al. 2007). Another issue often associated with the use of radiolabeled peptides is their high uptake and retention by the kidneys, which is a concern, particularly for radionuclide therapy because of the potential nephrotoxicity (Valkema et al. 2005; Imhof et al. 2011; Teunissen et al. 2005).
10.2.7 Development of Peptide-Based Radiopharmaceuticals
The synthesis of stable and well-defined peptides for use for targeted therapy is an important aspect of providing these tools for clinical application and involves a well-defined strategy and series of events (Okarvi 2004). It is initially essential to identify the enzyme or receptor molecular target (receptor) with relevance to human disease and identify for a potential tumor-specific peptide, either natural or synthetic. The endogenous ligand that displays high affinity for the corresponding receptor system must be identified, and the molecularly engineered synthesis of the peptide must be developed followed by attachment of a bifunctional chelating agent (BFCA) through a metabolic-resistant covalent bond. Adaptation of an appropriate radiolabeling procedure is required to permit high labeling efficiency to obtain a high-specific-activity product. It is crucial to choose a radiolabeling procedure that retains the receptor-binding affinity of the peptide. Screening by in vitro binding studies is often an important tool in this assessment. In vitro characterization includes studies such as binding of the radiopeptide with tumor cells, stability of chelated peptide in serum, receptor-binding affinity, internalization into the tumor cells, and dissociation from the tumor cells. These studies are critical for recognition of the potential peptide receptors and tumor types that are to be targeted with a specific peptide. Subsequent studies involve in vivo evaluation of the radiopeptide with respect to tumor-targeting properties using animal models. In order to assess the in vivo stability of the metal complex, evaluation in an appropriate animal model is necessary. After completion of these required preclinical and other tests, toxicological studies, and established radiopharmaceutical preparation, the radiopeptide is then assessed for efficacy in potential human studies involving only a small number of patients.
10.2.8 Preparation of Radiolabeled Peptides
Successful use of peptide-based targeted agents for targeted delivery of therapeutic radionuclides involves development of appropriate radiolabeling procedures. This requires suitable chelation chemistry for adequate sequestration of the radionuclide of interest, which usually is a metallic cation requiring appropriate chelation (i.e., 177Lu+3, 90Y+3, etc.). In this context, both the rate at which the metal complex forms and the rate of dissociation need to be considered. Cavity size of the bifunctional chelating agent (BFCA) should be compatible with the ionic radius of the radionuclide such that all of required donor groups can be properly aligned for optimal metal binding to provide high stability and limiting dissociation, i.e., thermodynamic stability and kinetic inertness. In recent years, a wide variety of BFCAs have been developed from well-established chelating agents, permitting rapid and convenient radiolabeling of peptides with different radionuclides. Structures of common acyclic and cyclic bifunctional chelating agents used for chelating radiometals in PRRT are shown in Fig. 10.4.
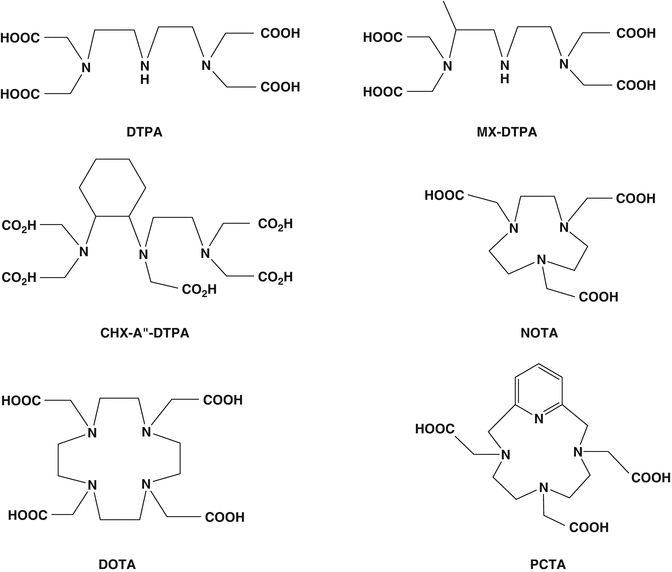
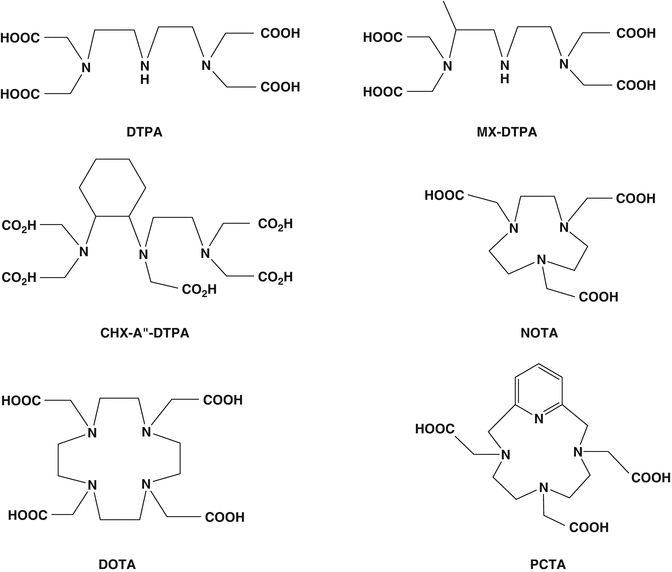
Fig. 10.4
Structures of typical acyclic and cyclic bifunctional chelating agents used for chelating radiometals
The major steps involved in the development of a peptide-based radiopharmaceutical are shown schematically in Scheme 10.1.
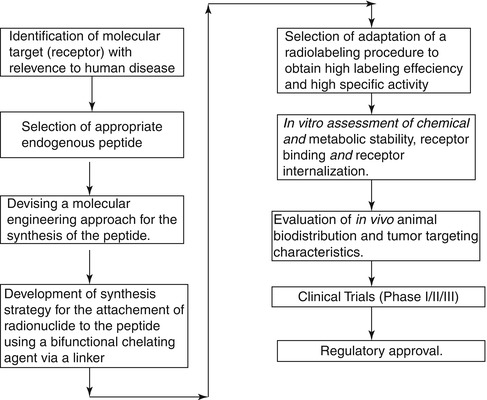
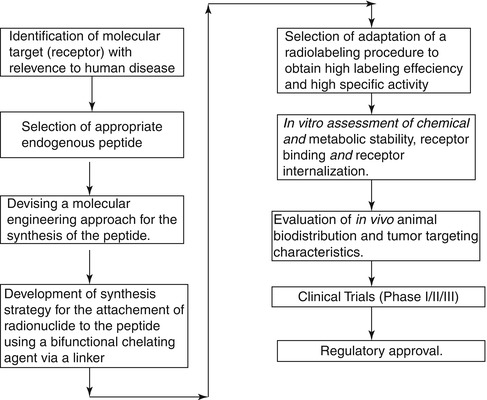
Scheme 10.1
Major steps involved in the development of a peptide-based radiopharmaceuticals
10.2.9 Radionuclides for Receptor-Mediated Peptide Therapy
Table 10.3 summarizes a number of important regulatory peptides and their analogs, which are of current interest for clinical PRRT application targeted to receptors overexpressed on tumors. As most of the peptides act through multiple peptide receptor subtypes, it is crucial that the peptide receptor subtype expressed by a given tumor corresponds to the subtype to which the radioligand used for PRRT binds with high affinity. The peptide analogs used as the targeting vector to deliver the radiation dose to the diseased site may function both as the receptor agonist or antagonist. An important feature of such agonists is that after binding to the receptor, the peptides or their radiolabeled analogs are internalized by receptor-mediated endocytosis, leading to an accumulation of the radioactive agonist within the tumor cell. On the other hand, peptide analogs, which function as receptor antagonists, have minimum or no internalization. Instead, once these agents bind to their cognate receptor, their function is blocked.
Table 10.3
Key regulatory peptides/analogs and their receptors overexpressed on tumors
Peptide | Number of amino acids | Receptor types/subtypes | Tumor expression |
---|---|---|---|
α-MSH | 13 | α-MSH-R | Melanomas |
Bombesin | 14 | BB1 (NMB-R), BB2 (GRP-R), BB3, BB4 | Prostate, breast, pancreas, gastric, colorectal, small-cell lung cancer |
CCK | 8, 33, 39, or 58 | CCK1, CCK2 | Medullary thyroid cancer, small-cell lung cancer, gastrointestinal stromal tumor, stromal ovarian cancer, astrocytomas |
GRP | 17 or 34 | CCK2 | |
LHRH | 10 | LHRH-R | Prostate, breast cancer |
Neurotensin | 13 | NTR1, NTR2, NTR3 | Small-cell lung cancer, colon, exocrine ductal pancreatic cancer, Ewing sarcoma, meningioma, astrocytoma, breast, prostate cancer |
RGD | 3 | Integrin αvβ3 | Glioma, breast, colon, lung cancer |
Somatostatin | 14 or 28 | SST1, SST2, SST3, SST4, SST5 | Neuroendocrine tumors (NETs) such as GEP-NET, carcinoids, paraganglioma, breast, brain, renal, small-cell lung cancer, medullary thyroid cancer |
8 | |||
Octreotide/octreotate | |||
Substance P | 11 | NK1, NK2, NK3 | Glial tumors (glioblastoma, medullary thyroid cancer), pancreas, breast, small-cell lung cancer |
VIP | 28 | VPAC1, VPAC2 | Adenocarcinomas of breast, prostate, stomach, and liver; neuroendocrine tumors |
Although it has been one of the most popular therapeutic radioisotopes for the past several decades, use of 131I in PRRT applications was not successful. Bakker et al. (1996) reported extensive radiolytic decomposition of octreotide after preparation of therapeutic dose of 131I labeled octreotide for somatostatin receptor targeted therapy (Bakker et al. 1996), which was attributed to the radionuclidic decay properties. Yttrium-90 and 177Lu are the most successfully used β−-emitting radionuclides, whereas the Auger electron-emitting radionuclide, 111In, has also been evaluated, although with relatively less success for PRRT. Very recently, alpha particle emitters such as 213Bi has been proposed for use (Norenberg et al. 2006; Cordier et al. 2010). The characteristics of the most commonly used radionuclides for PRRNT are summarized in Table 10.4.
Table 10.4
Characteristics of some key radionuclides proposed for PRRT
Radionuclide | Half-life | Emissions | Mean energy (keV) | Maximum tissue penetration range of particle | Source |
---|---|---|---|---|---|
Indium-111 | 2.81 days | Conversion electrons | 245 | 550 μm | Cyclotron |
Auger e | 25 | 10 μm | |||
γ-rays | 171, 245 | ||||
Yttrium-90 | 2.67 days | β−-particles | 934 | 12 mm | Generator |
Lutetium-177 | 6.65 days | β−-particles | 149 | 3 mm | Reactor |
γ-rays | 208 | ||||
Copper-67 | 2.58 days | β−-particles | 121 | 2–3 mm | Reactor/cyclotron |
γ-rays | 184 | ||||
Scandium-47 | 3.42 days | β−-particles | 143 | 3 mm | Reactor/cyclotron |
γ-rays | 159 | ||||
Terbium-161 | 6.90 days | β−-particles | 154 | 3 mm | Reactor |
γ-rays | 75 | ||||
Actinium-225 | 10.0 days | α-, β−-particles, γ-rays | Decay series | – | Accelerator |
Reactor | |||||
Bismuth-213 | 46 min | α-, β−-particles, γ-rays | Decay series | – | Generator |
10.3 Peptide Receptor Radionuclide Therapy (PRRT) for Neuroendocrine Tumors
Neuroendocrine tumors (NETs) represent a heterogeneous group of neoplasms which originate mainly from the gastroenteropancreatic tract (GEP NETs). The tumors include those arising from the endocrine cells within the respiratory and gastrointestinal tracts, known as “carcinoid” tumors. These tumors are classified as foregut, midgut, and hindgut, according to their presumed embryological origins. They are characterized by their endocrine metabolism and histological pattern. As opposed to other tumor entities, they range from well-differentiated, slow-growing tumors to poorly differentiated, highly invasive malignancies and may be functioning or nonfunctioning. NETs characteristically synthesize, store, and secrete a variety of peptides and neuroamines which may lead to clinical syndromes such as the carcinoid syndrome, the Zollinger–Ellison syndrome, or the Verner–Morrison syndrome (ENETS Consensus Guidelines 2008; Modlin et al. 2008). However, most GEP NETs are clinically silent until late presentation with metastases. Features such as neuroamine uptake mechanisms and/or specific receptors at the cell membrane, such as somatostatin receptors (SSTRs), provide the scope for the identification, localization, and therapy of NETs (Oberg 2002, 2004). Of the five major subtypes of SSTR which bind the 14-amino-acid peptide somatostatin and its high-affinity 28-amino-acid precursor, SSTR2 and SSTR5, are the subtypes most commonly expressed in NETs. However, there is considerable variation in SSTR subtype expression among the different tumor types and among tumors of the same type (de Herder et al. 2003). The majority of NETs express SSTRs and appear as ideal targets and provide scope for treatment with somatostatin analog (SST analog)-derived radiopeptides (Krenning et al. 1999; de Herder et al. 2003). The extraordinary affinity of these peptides for SSTRs together with the internalization of the receptor–peptide complex facilitates retention of the radiopeptide in receptor-expressing tumors, and at the same time their relatively small size facilitates rapid blood clearance. Because of the necessity to ensure the stability of the radiolabeled molecule, the radioisotope is bound to a BFCA which is covalently attached to the peptide. Structures of several key common DOTA-coupled somatostatin analogs are depicted in Fig. 10.5.
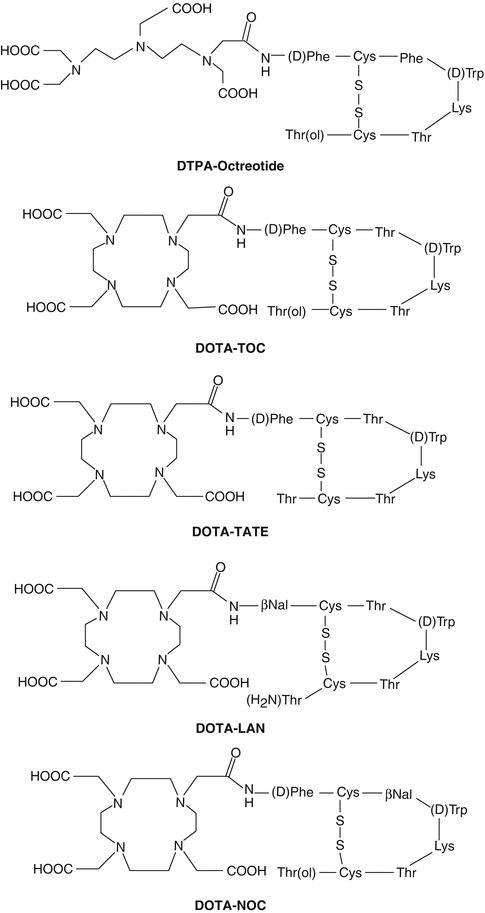
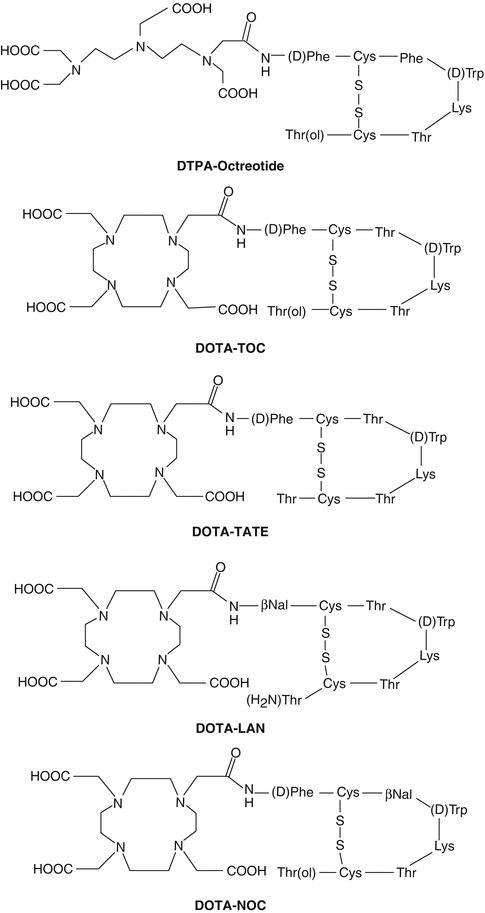
Fig. 10.5
Structures of common DTPA/DOTA-coupled somatostatin analogs
Common DOTA-coupled, somatostatin-based radiopharmaceuticals are 90Y-DOTA0-Tyr3-octreotide (90Y-DOTATOC), 90Y-DOTA-lanreotide, and 177Lu-DOTA-Tyr3-Thre8-octreotide (177Lu-DOTATATE). The DOTATATE derivative exhibits the highest affinity to SSTR2 and hence widely used as a vector for targeted therapy. On the other hand, DOTA-lanreotide possesses the lower affinity to SSTR2 although demonstrating considerable SSTR5 affinity (Krenning et al. 2005). Factors that determine the amount of uptake of radiolabeled SST analogs include stability of the radioligand, density of SSTR expression on the tumor, type of SSTRs expressed by the tumor, affinity of the radioligand for the SSTRs, efficiency of SSTR-mediated internalization, and the mass of the administered peptide. Several radiolabeled SST peptide analogs which are currently in clinical use for treatment of patients with SST receptor-expressing tumors are described below.
10.3.1 PRRT Studies with [111In-DTPA]octreotide
Investigator in the mid- to late-1990s used [111In- DTPA]octreotide for PRRT since this approved radiopharmaceutical was already in use for diagnostic imaging. Since 111In is an Auger electron-emitting radionuclide, the goal was to increase the administered dose to assess possible therapeutic effects. While the use of high activity levels of [111In-DTPA0]octreotide in patients with metastasized neuroendocrine tumors was initially encouraging with regard to symptom relief, tumor size regression was unsatisfactory (Valkema et al. 2002; Anthony et al. 2002). The major impediments for expanded use of 111In-coupled peptides are the small particle range of the particle and consequently short tissue penetration (~10 μm) and radiotoxicity (Kaltsas et al. 2005; Kwekkeboom et al. 2005).
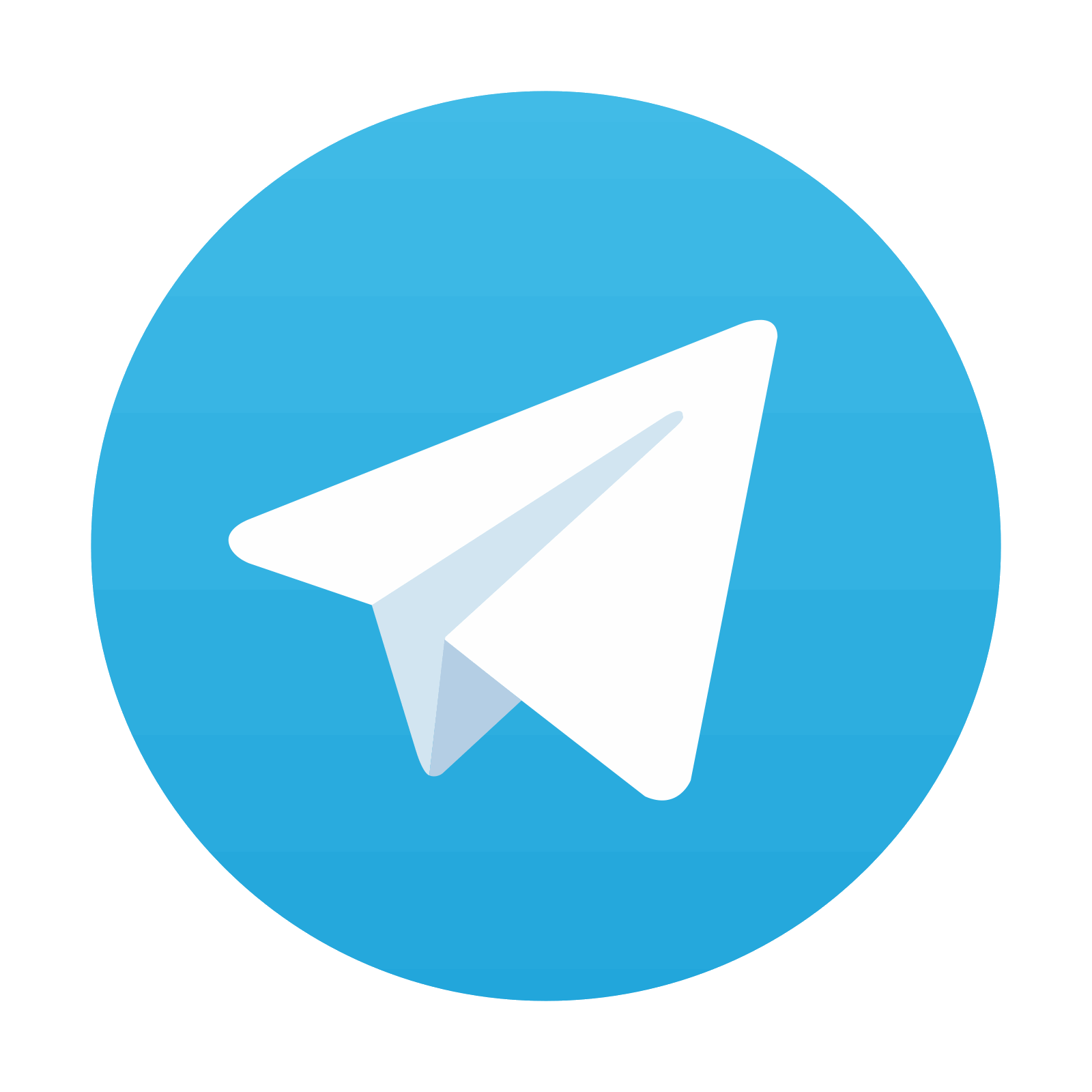
Stay updated, free articles. Join our Telegram channel
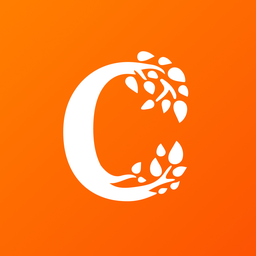
Full access? Get Clinical Tree
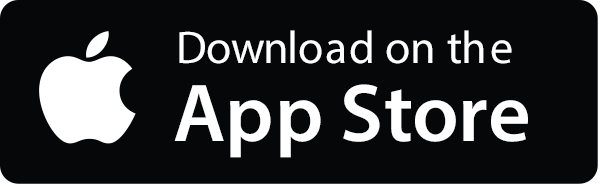
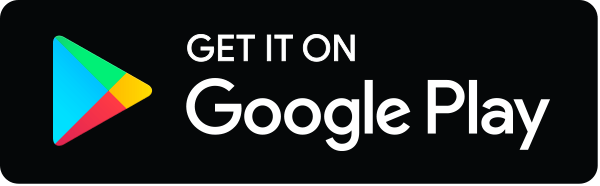