- •
PAD is prevalent and associated with high morbidity and mortality but is frequently underdiagnosed.
- •
Conventional approaches to diagnosis, including ABI and angiography (invasive and noninvasive), can only assess the extent and severity of macrovascular atherosclerosis.
- •
There is growing recognition that microvascular abnormalities in PAD contribute to patient symptoms and portend adverse clinical outcomes, including wound healing, infection, and limb amputation.
- •
Microvascular abnormalities and associated skeletal muscle remodeling are poorly captured by standard diagnostic modalities.
- •
Radionuclide perfusion imaging with SPECT and PET provide accurate, quantitative assessments of tissue perfusion that complement angiographic data. As such, they can be used to identify potential therapeutic benefit from revascularization and medical management, including supervised exercise therapy.
- •
Radionuclide imaging using targeted radiotracers are emerging as potential powerful tools for assessing novel cell and gene therapies for PAD.
Epidemiology and the natural history of peripheral arterial disease
Over the last several decades, cardiovascular imaging has expanded to incorporate peripheral vascular disease, which affects the arterial, venous, and lymphatic systems. The primary focus of this chapter will be on arterial disease of the lower extremities, a progressive atherosclerotic process that causes stenosis and occlusion.
In 2010, peripheral arterial disease (PAD) affected 200 million people worldwide with a prevalence that increased with age and in association with diabetes mellitus (DM). PAD is associated with an increased risk for cardiovascular disease and is the third leading cause of atherosclerotic cardiovascular comorbidity after coronary artery disease (CAD) and stroke. The increased morbidity and mortality risks are due to downstream clinical manifestations of intermittent claudication, nonhealing ulcers, the need for limb amputation, associated increased risk for cardiac events, and death. Patients with PAD experience a diminution in quality of life, largely attributable to impaired ambulatory physical function.
Despite the high prevalence and significant effect on patient quality of life and survival, PAD is frequently underdiagnosed. The challenges in identification of PAD are due to (1) underrecognition of symptoms, (2) the prevalence of asymptomatic or variable presentation of PAD, and (3) limitations in standard noninvasive diagnostic modalities, such as ankle-brachial index (ABI), ultrasound (US), computed tomography (CT), and magnetic resonance imaging (MRI). These standard imaging modalities have utility in assessing the macrovascular circulation, particularly for inflow disease and in the absence of extensive calcification. As such, these techniques are used to assess patency of the conduit lower-extremity arteries and surveillance after lower-extremity revascularization.
Similar to our evolving understanding of CAD, an expanded understanding of PAD beyond just macrovascular disease requires assessment of the microvascular circulation in the lower extremities, along with pairing of functional and anatomic assessments of PAD, characterization of the biology of the atherosclerotic plaque, a measure of the angiogenic and arteriogenic response, and assessment of the metabolic health of the downstream skeletal muscle. With their ability to image both perfusion and biologic processes, radionuclide imaging techniques offer promise to improve our understanding of pathophysiology, help with diagnosis, and guide in the treatment of PAD. Given that these emerging techniques are in development and not yet part of clinical practice, clinical insights are embedded in the text to highlight challenges in our current standard for assessment of PAD and opportunities that may exist for radionuclide techniques.
Compartments of peripheral arterial disease: Macrovasculature, microvasculature, angiosomes, and collateral vessels
In patients with PAD, reduced physical function, development of wounds, and tissue loss are because of insufficient blood flow and perfusion, resulting from impaired oxygen and nutrient supply to the lower extremity skeletal musculature. Reduced oxygen supply is traditionally attributed to progressive narrowing of the large arteries from atherosclerosis. The macrovascular arterial system is divided into inflow (conduit) arteries (i.e., aorta and iliac branches) and outflow arteries. The outflow arteries include the arteries above-the-knee (i.e., the superficial femoral artery) and the below-the-knee vasculature (i.e., the popliteal artery and infrapopliteal arteries—anterior tibial, posterior tibial, and peroneal arteries).
The angiosome concept of foot perfusion is based on anatomic studies of arterial circulation for planning of revascularization and/or amputation. Angiosomes are three-dimensional (3D) blocks of tissue that consist of skin, subcutaneous tissue, fascia, muscle, and bone. They are supplied by specific upstream infrapopliteal arteries (anterior tibial, posterior tibial, and peroneal arteries), which, in turn, supply downstream vascular runoff territories in the foot. There are six foot angiosomes, including three that originate from the posterior tibial artery, one from the anterior tibial artery, and two from the peroneal artery ( Fig. 31.1 ).
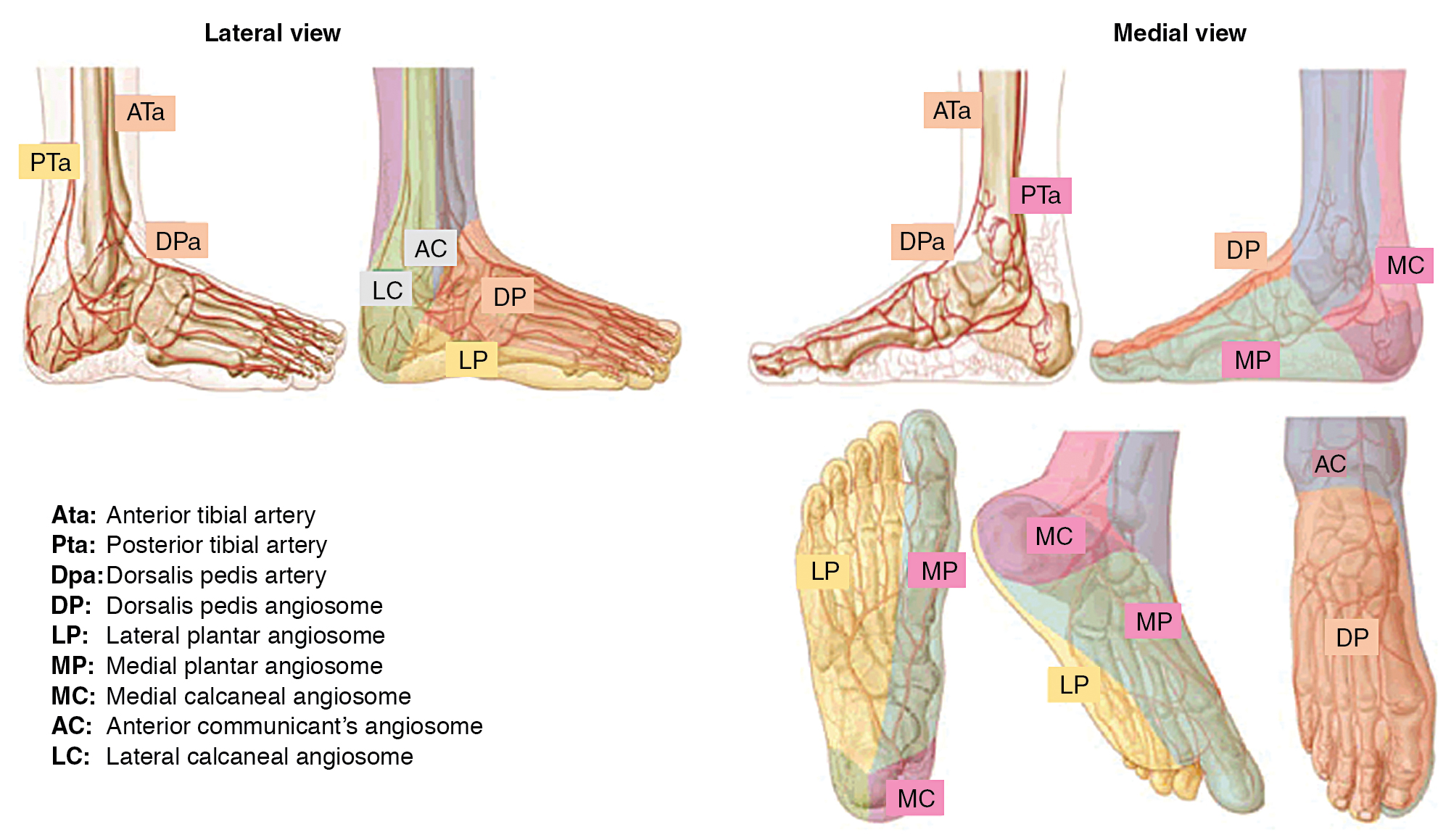
Critical limb ischemia (CLI) with complicating ischemic and neuroischemic foot ulcers is more common in patients with DM. The construct of angiosomes is applied in patients with DM and CLI to predict healing after revascularization because angiosome-directed revascularization has been associated with improvement in wound healing and limb salvage. Revascularization planning is informed by identification of the macrovascular level of stenosis, which is often multilevel. Although multivessel infrapopliteal disease is common, complete revascularization of all three infrapopliteal vessels is not always possible and is associated with poor long-term patency, whereas angiosome-directed revascularization allows for targeting of specific anatomic regions ( Fig. 31.2 ).
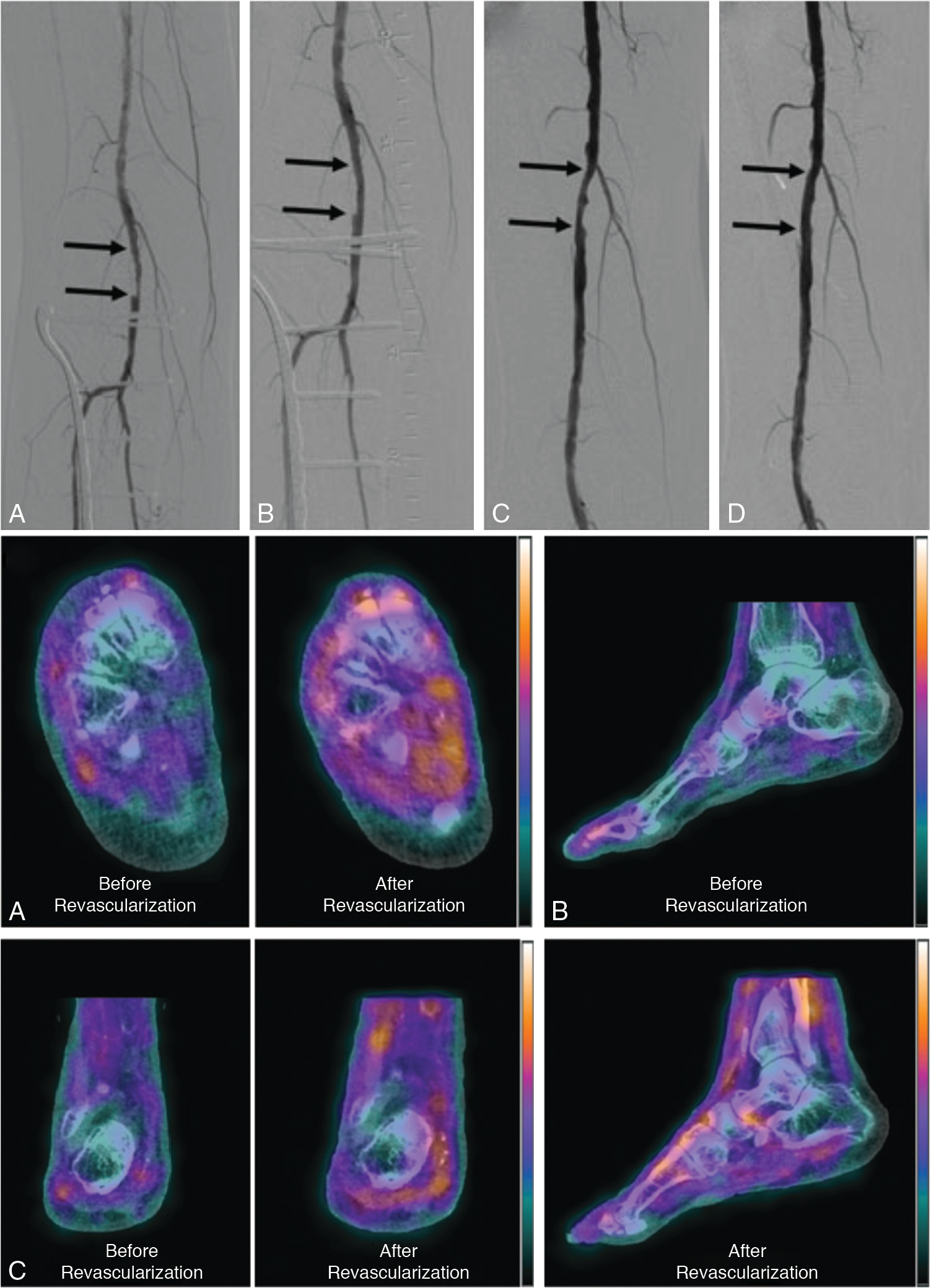
Collateral reserve is an important natural “rescue system” to maintain perfusion in a diseased angiosome. The corollary is that collateral depletion, as has been documented in patients with DM and end-stage renal disease, may be operative in CLI. In patients with diabetic end-artery disease, so-called patchy atherosclerosis occurs, along with septic thrombosis and loss of small collaterals. As such, there is growing recognition that a dysfunctional microvascular system and loss of capillary density plays a critical role in abnormal perfusion to skeletal muscle. The small pedal arteries (1 to 2 mm in diameter) and the microvasculature (<100 μm) account for limb symptoms in patients with and without macrovascular PAD ( Fig. 31.3 ). It has been suggested that in patients with DM, the occurrence of the so-called diabetic foot is related to a combination of distal atherosclerotic macroangiopathy and an impairment of microcirculatory function, which is associated with neuropathy, reduced immune function, and local sepsis. As such, impairment in the microcirculation plays a significant role in lower-extremity ulceration and affects wound healing.
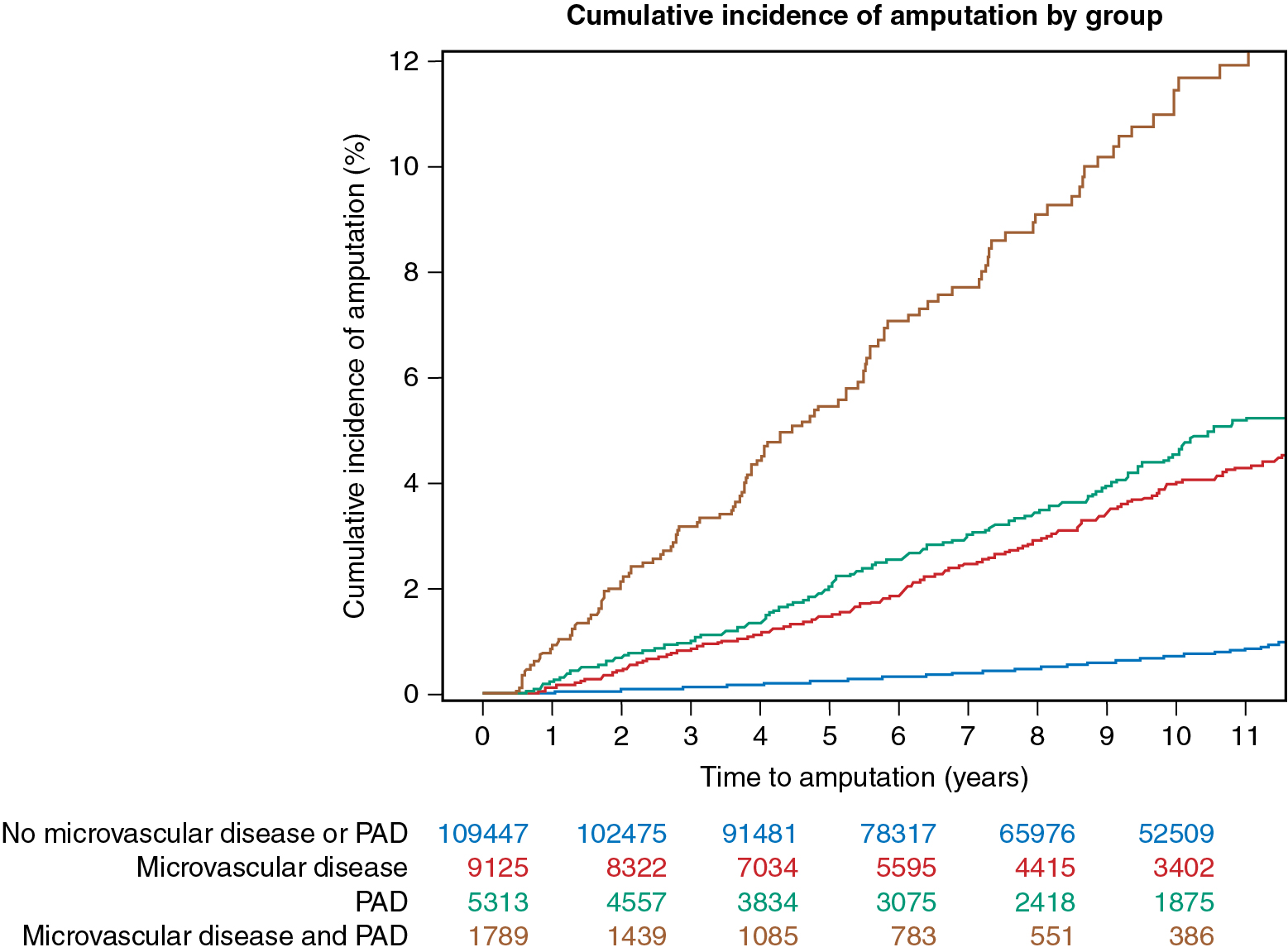
Microvascular dysfunction represents a complex number of factors that contribute to lower-extremity disease in those with DM. Contributing factors include peripheral autonomic neuropathy, regional sepsis, endothelial dysfunction with associated atherosclerosis, and dysfunctional vascular smooth muscle cells. Microvascular dysfunction is worsened by autonomic dysfunction, with loss of autoregulatory function, an impaired hyperemic and inflammatory response, dysfunctional vasomotion, increased arteriovenous shunting, impaired oxygen diffusion, and leukocyte migration. These regulated biologic systems become dysfunctional and result in poor wound healing in diabetic patients. Radionuclide imaging offers promise as an assessment tool of the microvascular system and perfusion reserve ( Fig. 31.4 ).
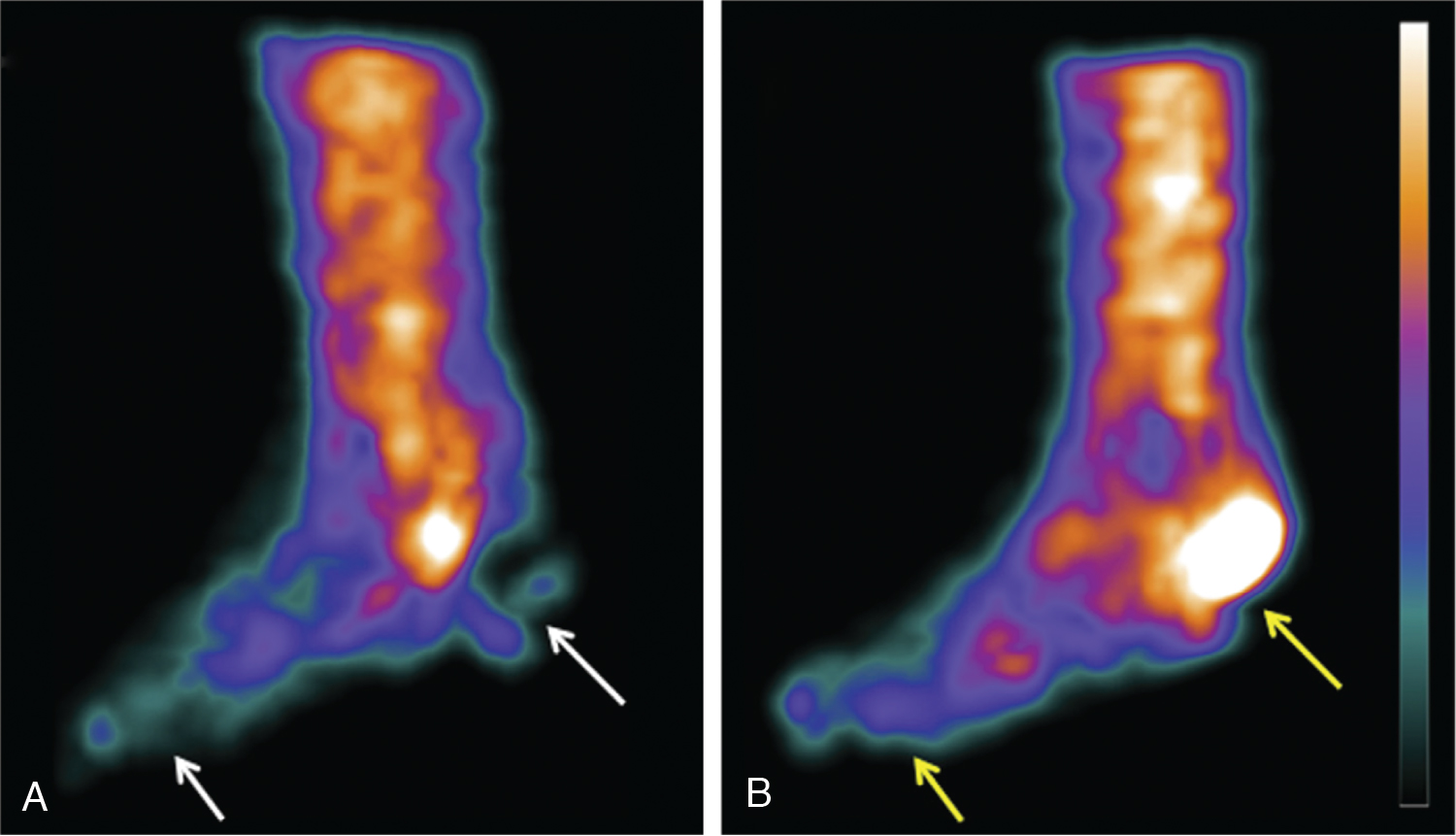
Standard noninvasive evaluation of peripheral arterial disease
Standard noninvasive diagnostic modalities of PAD are best understood in the context of the anatomic classifications of PAD and primarily assess macrovascular arterial stenosis and occlusion. A brief summary of standard noninvasive diagnostic modalities is presented with categorization of modalities by (1) anatomic versus physiologic assessment, (2) macrovascular versus microvascular assessment, and (3) rest versus provocation assessment ( Table 31.1 ).
Diagnostic Modality | Categorization | Advantages | Limitations |
---|---|---|---|
Ankle-brachial index (ABI) |
|
|
|
Exercise ABI |
| Exercise-provoked drop in ABI to identify proximal inflow disease missed by resting ABI | |
Segmental pressure |
| Provides anatomic localization of disease | Overestimation in medial calcification, common in DM, ESRD, advanced age |
Pulse volume recording (PVR) |
|
| Lacks quantification |
Toe brachial index (TBI) |
|
|
|
Transcutaneous oxygen pressure (TcPO 2 ) |
| Measure of superficial tissue viability |
|
Duplex ultrasonography |
|
|
|
Computed tomography angiography (CTA) |
|
|
|
Magnetic resonance angiography (MRA) |
|
| Limited spatial resolution |
|
|
|
|
Radionuclide imaging techniques offer potential to overcome many of the limitations of standard noninvasive assessment of PAD. Although positron emission tomography (PET) has higher sensitivity than single photon emission computed tomography (SPECT) for targeted molecular imaging and lower levels of ionizing radiation through the use of isotopes with short half-lives, SPECT systems are more readily available. Unfortunately, SPECT and PET for molecular imaging have lower spatial resolution than CT and MRI. Given this limitation, hybrid imaging systems (PET/CT, SPECT/CT, PET/MRI) allow for the pairing of high-sensitivity physiologic images with quantification of radionuclide uptake in anatomic regions of interest and allow for topographic localization, attenuation correction, and correction for partial volume effects. Additionally, new targeted radiotracers can assess specific biologic processes that are attendant to the biologic underpinnings of PAD, such as atherosclerosis, angiogenesis, inflammation, thrombosis, and ischemia ( Table 31.2 ).
Application | Modality | Radionuclide | Biologic Target | Application |
---|---|---|---|---|
Skeletal muscle perfusion and blood flow | SPECT | 201 Thallium (Tl) | Clinical | |
99m Technetium (Tc)-sestamibi | Clinical | |||
99m Tc-tetrofosmin | Clinical , | |||
PET | 15 O-water | Clinical , | ||
C 15 O 2 | Clinical | |||
15 O 2 | Clinical , | |||
13 N-ammonia | Preclinical: mouse | |||
Skeletal muscle angiogenesis | SPECT | 99m Tc-NC100692 | ανβ3 integrin | Preclinical: mouse |
111 Indium (In)-VEGF121 | VEGF receptor | Preclinical: rabbit | ||
125 1-c(RGB(I)yV) | ανβ3 integrin | Preclinical: mouse | ||
PET | 76 Br-nanoprobe | ανβ3 integrin | Preclinical: mouse | |
68 Ga-NOTA-RGB | ανβ3 integrin | Preclinical: mouse | ||
64 Cu-DOTA-CANF-comb | NPR-C | Preclinical: mouse | ||
64 Cu-DOTA-VEGF 121 | VEGF receptor | Preclinical: mouse | ||
64 Cu-NOTA-TRC105 | CD105 | Preclinical: mouse , | ||
Peripheral atherosclerosis | PET | 18 F-FDG | Macrophages | Clinical |
68 Ga-Dotate | Somatostatin receptor-2 | Preclinical | ||
18 F-methylcholine ( 18 F-FMCH) | Macrophage cell membranes | Preclinical: mouse | ||
11 C-PK11195 | Translocator protein (TSPO) on macrophage | Preclinical: mouse | ||
18 F-Galacto-RGB | ανβ3 integrin | Preclinical: mouse Clinical | ||
18 F-Fluciclatide | ανβ3 integrin | Preclinical | ||
18 F-NaF | Microcalcification | Clinical: aorta, carotid, femoral | ||
11 C-acetate | Fatty Acid Synthesis | Clinical; aorta, carotid, iliac | ||
64 Cu-DOTA-CANF | C-type atrial natriuretic factor | Preclinical: rabbit | ||
99m Tc-PBMC | Peripheral blood mononuclear cell | Clinical | ||
18 F-fluoromisonidazole (FMSO) | Hypoxia | Clinical | ||
18 F-HX4 | Hypoxia | Preclinical | ||
64 Cu-ATSM | Hypoxia | Preclinical | ||
Skeletal muscle metabolism | PET | 18 F-FDG | Glucose analog | Clinical |
18 F-Fluoro-4-thia-octadec-9-enoate ( 18 F-FTO) | Oleate-free fatty acid | Clinical |
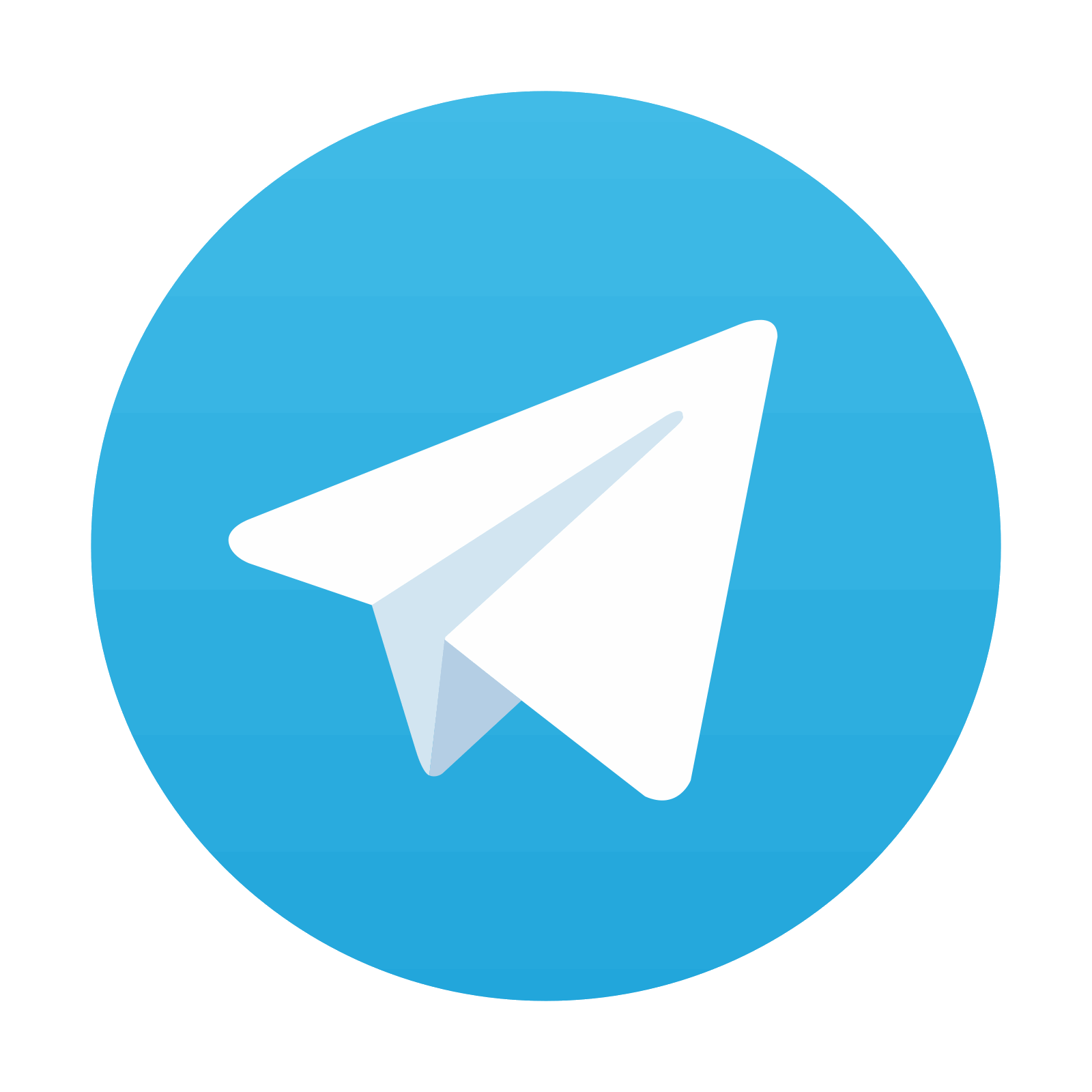
Stay updated, free articles. Join our Telegram channel
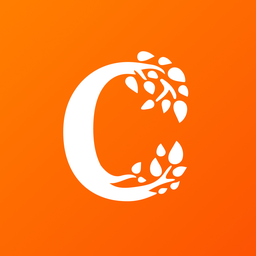
Full access? Get Clinical Tree
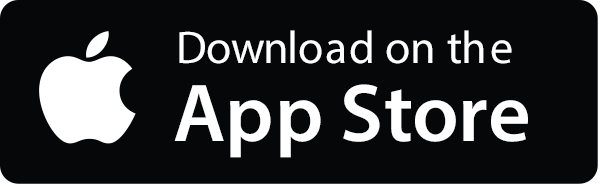
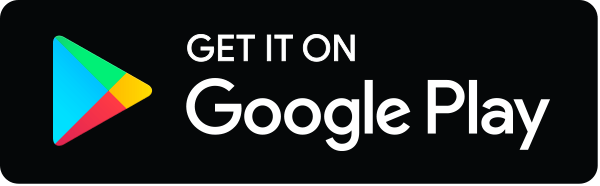
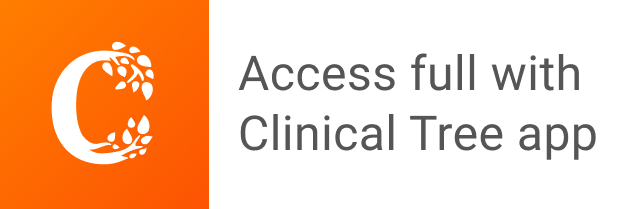