Pulmonary Scintigraphy
David K. Shelton
Meena Kumar
Although CT angiography (CTA) has taken a central role in diagnosing pulmonary embolism (PE), ventilation–perfusion (V/Q) scans remain an important imaging test. A scintigraphic lung scan is a physiologic map that evaluates the primary functions of the lung, pulmonary vasculature perfusion, and segmental bronchioalveolar tree ventilation. Most commonly, V/Q scans are used to evaluate patients suspected of having PE. In an attempt to provide more accurate results, the criteria for interpreting V/Q studies have been constantly revised. Different schema that compare defects present on the perfusion scan with those found on the ventilation scan and/or chest x-ray (CXR) have been developed in order to estimate the probability of PE. This chapter describes radiopharmaceuticals used, examination technique, imaging protocols, and criteria for the interpretation of V/Q scans.
Anatomy and Physiology
Understanding the segmental anatomy of the lungs (Fig. 55.1) is vital to the interpretation of lung scans. The three-dimensional location of ventilation or perfusion defects must be individually determined and correlated with the segmental or subsegmental anatomy of the lung. PE will have a segmental or a subsegmental distribution pattern, usually peripheral and wedge-shaped in nature.
Although pulmonary ventilation occurs primarily via the branching bronchial system, other pathways exist by which distal alveoli can be aerated. The pores of Kohn connect adjacent alveoli, and the canals of Lambert connect alveoli with respiratory, terminal, and preterminal bronchioles. These canals and pores permit collateral ventilation of alveoli whose conducting airways have become blocked. Collateral air drift is dynamic and is mediated by neurohormonal control that can be altered by pathologic events, atmospheric/alveolar gas tension, and drugs.
Both ventilation and pulmonary blood flow demonstrate marked gravitational effects. When a patient is in an upright posture, the gradient for blood flow is from the apices to the lung bases; the apex receives only one-third of the blood volume that the base receives. A corresponding ventilation gradient exists when the patient sits upright. Since the intrapleural pressure is greater at the bases, the differential negative intrapleural pressure at the apices causes the alveoli at the apices to remain more open at expiration than the alveoli at the lung bases. Therefore, the basilar alveoli undergo greater respiratory cycle changes in size. This results in greater gas exchange occurring in the base and greater oxygen tension in the apices. On average, ventilation at the base is 1.5 to 2 times that of the apex. When a patient is supine, the ventilation gradient shifts from superioinferior to anteroposterior, and perfusion is increased to the dependent posterior portions of the lungs (1).
Normally, capillary perfusion and alveolar ventilation are matched in order to maximize gas exchange. Diseases that produce localized hypoxia invoke autoregulatory mechanisms that divert blood flow away from the hypoxemic pulmonary segments. These dynamic changes prevent nonventilated lung segments from being perfused. Conversely, localized hypoperfusion rarely induces localized bronchoconstriction. Primary vascular disorders such as PE, if unassociated with parenchymal consolidation or pulmonary infarction, usually have normal ventilation (1). Thus, an anatomic perfusion deficit with normal ventilation is referred to as a V/Q mismatch and is the hallmark of PE diagnosis.
Ventilation Lung Scan
Radiopharmaceuticals
Xenon-133. Xe-133 is a radioisotope widely used to perform ventilation lung scans. A noble gas produced by fission of U-235 in a nuclear reactor, Xe-133 has a half-life of 5.3 days, and is a beta emitter. The principle photon energy is 81 keV resulting in significant attenuation effects. Xe-133 ventilation scans should be performed before perfusion lung scans because Compton scatter from the higher energy Tc-99m macroaggregated albumin (MAA) (140 keV) down-scatters into the region of the 81-keV photopeak of the Xe-133 and would thus interfere with ventilation images. The usual adult dose of Xe-133 for a ventilation scan is 10 to 20 mCi (370 to 540 MBq).
Xenon-127. Xe-127 is a cyclotron-produced isotope with a physical half-life of 36.4 days and principle photon energies of 203 keV, 172 keV, and 365 keV. Because it has higher
energy photons, Xe-127 ventilation scans can be performed, if needed after the perfusion scan, since down-scatter image deterioration is not a significant problem. Unfortunately, because Xe-127 is cyclotron-produced, it is both expensive and of limited availability. The usual adult dose is 8 to 15 mCi (296 to 555 MBq).
energy photons, Xe-127 ventilation scans can be performed, if needed after the perfusion scan, since down-scatter image deterioration is not a significant problem. Unfortunately, because Xe-127 is cyclotron-produced, it is both expensive and of limited availability. The usual adult dose is 8 to 15 mCi (296 to 555 MBq).
Krypton-81m. Kr-81m is the other noble gas used for ventilation scans. Having an extremely short half-life of only 13 seconds, Kr-81m is produced from a Rb-81 to Kr-81m generator. Kr-81m decays by isomeric transition and has a photon energy of 191 keV. The higher energy allows the ventilation scan to be acquired, if needed, after an abnormal perfusion scan. Unfortunately, the generator is expensive and therefore Kr-81 is limited in use. The usual adult dose is 10 to 20 mCi (370 to 740 MBq).
Technetium-99m Aerosols. Ventilation scans can be performed using aerosolized rather than gaseous agents. Radioisotope-labeled aerosols are produced by nebulizing radiopharmaceuticals into a fine mist that is inhaled. Tc-99m diethylenetriaminepentaacetic acid (DTPA) is the most commonly used radioaerosol. The advantages of Tc-99m aerosols are that they are widely available, inexpensive, and have a 140-keV photopeak ideal for gamma camera imaging. The nebulizer-produced mist is passed through a settling bag, which traps larger particles. The mist is delivered to the patient via a nonrebreathing valve and is inhaled. The process is inefficient; only 2% to 10% of the aerosolized radioisotope is deposited within the lungs. Of the 30 mCi of nebulized Tc-99m DTPA, only 1 to 2 mCi are actually deposited within the lungs.
The site of deposition of the aerosolized particles depends on the size of the inhaled particle. The larger the particle is, the greater the gravitational effect, which results in more central deposition. Particles larger than 2 microns localize in the trachea and pharynx. Current aerosol nebulizers can produce microaerosols of less than 0.5 microns. Thus, microaerosol particles are small enough to reach the distal tracheobronchial tree, and reflect regional ventilation. Patients with narrowed airways caused by asthma, bronchitis, or chronic obstructive pulmonary disease (COPD) have more central deposition of the particles than normal patients because of airway turbulence. This results in poor visualization of the peripheral lung fields. The deposited Tc-99m DTPA is absorbed across the alveolar membrane with a clearance half-life of 60 to 90 minutes. The half-life is approximately 20 minutes shorter in tobacco smokers, owing to their increased alveolar permeability.
Dosimetry. The critical organ for Xe-133 is the trachea, which receives a dose of 0.64 rad/mCi. The lung dose is 0.01 to 0.04 rad/mCi whereas the whole-body absorbed dose is 0.001 rad/mCi.
For Tc-99m aerosols, the lungs receive an absorbed dose of 0.1 rad/mCi, the bladder wall a dose of 0.18 rad/mCi, and the entire body a dose of 0.01 rad/mCi.
Ventilation Scan Technique
Ventilation scanning using radioactive gases requires special equipment to prevent leakage of the gas into the imaging room. Gas delivery systems consist of a shielded spirometer, oxygen delivery system, and a xenon charcoal trap, to capture most of the exhaled xenon. Because xenon is heavier than air, loose xenon pools at floor level, and thus the room should be well ventilated and have a negative pressure flow.
Xenon-133 Ventilation Scanning. The patient is initially fitted with an airtight face mask. While the patient takes a maximal inspiration, Xe-133 is injected into the mask intake tubing. The patient is instructed to hold his or her breath as long as possible. A posterior projection 100,000-count first-breath image of the lungs is then obtained. The ventilation system is then switched so that the patient rebreathes the air–Xe-133 mixture. After 5 minutes of rebreathing, a posterior 100,000-count equilibrium image is obtained. The distribution of Xe-133 activity on the equilibrium image represents aerated lung volume. The ventilation system is then readjusted so that the patient breathes in fresh air and exhales the Xe-133 mixture into the trap. Serial posterior 30-second washout images are obtained over a 5-minute interval. The Xe-133 normally washes out of the lungs within 3 to 4 minutes (2). Because the lung bases are better ventilated than the apices, the Xe-133 washes out of the bases faster than at the apices in a normal patient. If possible, all images should be performed with the patient in an upright position.
Xenon-127 ventilation scanning is performed in the same manner as Xe-133 ventilation lung scans. The Xe-127 ventilation scan needs to be performed only if the perfusion scan is abnormal.
Krypton-81m Ventilation Scanning. The high-photon energy of Kr-81m allows ventilation scans to follow perfusion scans. Immediately after each perfusion image and without moving, the patient inhales Kr-81m and the corresponding ventilation image is obtained. This process is repeated until ventilation and perfusion images are obtained in all six matching positions.
Technetium-99m Aerosol Ventilation Scanning. The patient inhales the nebulized aerosol while in the supine position to avoid the normal apex-to-base gravity gradient. After inhaling the Tc-99m aerosol for 3 to 5 minutes, the patient sits upright and is imaged in the same projections as for the perfusion lung scan. The exhaled aerosol is trapped in a filter that is stored until decay is sufficient for safe disposal.
A Tc-99m aerosol ventilation lung scan can be performed either before or after the perfusion lung scan. If the perfusion scan is performed first, a small dose (0.5 mCi) of Tc-99m MAA is used with a large dose (30 mCi) of Tc-99m DTPA. If the ventilation scan is performed first, 5 to 10 mCi of Tc-99m DTPA and 5 mCi of Tc-99m MAA are administered.
Perfusion Lung Scan
Radiopharmaceuticals
Perfusion lung scanning is based on the principal of capillary blockade. Particles slightly larger than the pulmonary capillaries (>8 μ) are injected intravenously and travel to the right heart where venous blood is uniformly mixed. Radiolabeled particles in the pulmonary arterial blood pass into the distal pulmonary circulation. Because the radioactive particles are larger than the capillaries, they lodge in the precapillary arterioles. Their distribution in the lung reflects the relative blood flow to pulmonary segments. Pulmonary segments with decreased or absent blood flow show diminished radioactivity.
Tc-99m macroaggregated albumin (MAA) is the radiopharmaceutical used to perform most perfusion lung scans. MAA is prepared by heat denaturation of human serum albumin. The MAA particles are irregularly shaped molecules with size range and number of particles in commercially available kits tightly controlled. Most particles are in the 20- to 40-micron size range with 90% of the particles between 10 and 90 microns. Particles larger than 150 microns should not be injected because they can obstruct arterioles. The size and number of particles in a kit are checked by counting a sample volume in a light microscopy hemocytometer. Tc-99m MAA is prepared by adding Tc-99m pertechnetate (Tc-99mO4) to the MAA kit. The MAA leaves the lungs by breaking down into smaller particles that pass through the alveolar capillaries into the systemic circulation where they are removed by the reticuloendothelial system. The biological half-life of MAA particles in the lung is 2 to 9 hours. The physical half-life of Tc-99m MAA is 6 hours.
A minimum of 60,000-Tc-99m MAA particles must be injected to ensure reliable count statistics and image quality. Typically, 200,000 to 500,000 particles are injected and less than 0.1% of capillaries are temporarily and safely occluded. However, several types of patients should receive a reduced number of particles during a perfusion scan. Patients with pulmonary hypertension and right to left shunts should be given only 100,000 particles. Children should also be injected with only 100,000 particles because they have fewer pulmonary arterioles. To perform reduced count imaging, each perfusion view is imaged for a longer time interval allowing for nearly equivalent count statistics. Alternatively, the kit can be reconstituted with higher than usual Tc-99m activity per particle. The normal 5-mCi dose can be administered but with fewer particles. Contraindications to perfusion lung scanning include severe pulmonary hypertension and allergy to human serum albumin products.
Dosimetry. The normal adult dose is 3 to 5 mCi (111 to 185 MBq). The lung is the critical organ and receives an absorbed dose of 0.15 to 0.5 rad/mCi. The whole body and gonadal absorbed dose are 0.15 rad/mCi.
Perfusion Scan Technique
The syringe containing the Tc-99m MAA should be gently agitated prior to injection to resuspend all particles. The patient is injected in the supine position while taking slow, deep breaths to minimize the pulmonary perfusion gravitational gradient (2). Blood should not be drawn into the syringe because aspirated blood may form clots, which become labeled by the Tc-99m MAA. Injection of clumped Tc-99m MAA particles or labeled clot can result in multiple small focal hot spots scattered through the lungs.
The patient is usually imaged in the upright position using a large field of view, high-resolution gamma camera. Images (500,000 counts) are obtained in the anterior, posterior, right lateral, left lateral, right posterior oblique, left posterior oblique, right anterior oblique, and left anterior oblique positions. Supplemental or decubitus views can be added to clarify findings on the standard views.
V/Q Scans
Indications. The most common indication for V/Q scans is the diagnosis of suspected PE. This examination has also been used to monitor pulmonary function of lung transplants, to provide preoperative estimates of lung function in lung carcinoma patients in whom pneumonectomy is planned (split lung function study), to evaluate right to left shunts, and to conduct serial assessment of inflammatory lung disease. The CXR should be evaluated prior to obtaining a V/Q scan. Infiltrates, effusions, pulmonary edema, or pneumothorax may explain sudden respiratory deterioration and eliminate the need for a V/Q scan.
CT Angiography Versus Ventilation/Perfusion Scans. There are situations in which V/Q scan should be the first option to diagnose PE and times when CTA should be the first option. Pulmonary angiography is rarely needed anymore. The sensitivity and accuracy of CTA have increased with the use of thin-cut helical CT and multidetector CT (MDCT) (Fig. 55.2). It should be considered when the patient is in the ICU, has an abnormal CXR, high clinical probability for PE, or a relative contraindication for anticoagulation. V/Q scan is highly sensitive and avoids the use of iodinated contrast. It should be considered when the clinical probability is low, the CXR is normal, and when the patient has a relative contraindication for iodinated contrast.
![]() Figure 55.2. CT Pulmonary Angiogram With MDCT. Scan demonstrates multiple, bilateral pulmonary emboli (arrows), and a left pleural effusion. |
Physiologic changes in pregnancy often mimic PE, making clinical diagnosis unreliable and resulting in diagnostic imaging. Both V/Q and CTA expose the mother and fetus to radiation and potential latent carcinogenic risks. However, the risks of undiagnosed PE or inappropriate anticoagulation outweigh any risk from diagnostic imaging. The fetus’ radiation dose is higher from V/Q (640 to 800 μGy) than from CTA (3 to 131 μGy) according to some authors, whereas other authors feel that the fetal dose is comparable. There is no consensus regarding which study is the first choice during pregnancy, and thus the clinician should consider clinical and CXR findings, radiation dose, and patient concerns when deciding on the appropriate diagnostic imaging (3). However, a common recommendation is to key on the CXR. If the CXR is normal, one can start with the V/Q scan. If the CXR is abnormal, CTA should be the first choice.
Regarding radiation dose to the breast, a 4-slice CT scan will deliver 20 to 60 mSv. For 64-slice CT, the breast radiation dose is 50 to 80 mSv. For V/Q scan, the breast radiation is 0.28 to 0.9 mSv. Thus, the radiation dose to the breast is 65 to 250 times higher for CTA than for V/Q scan (4).
Normal ventilation scans (Fig. 55.3A) have homogeneous radiopharmaceutical distribution throughout all lung fields on all three phases of the scan, initial breath, equilibrium, and washout. A subtle base-to-apex gradient may be seen
because more lung parenchyma is located at the base than at the apex. The first-breath Xe-133 image is often grainy because it has relatively poor count statistics. However, it still reflects regional lung volume. The equilibrium images have greater activity and will fill in areas of restricted lung disease. The washout phase of the study demonstrates rapid clearance of the Xe-133 from the lungs. Normal half-time for xenon washout is less than 1 minute. Washout is complete within 3 minutes. Retention (trapping) of xenon in the lungs in a focal or in a diffuse pattern is an indication of obstructive lung disease (Fig. 55.4).
because more lung parenchyma is located at the base than at the apex. The first-breath Xe-133 image is often grainy because it has relatively poor count statistics. However, it still reflects regional lung volume. The equilibrium images have greater activity and will fill in areas of restricted lung disease. The washout phase of the study demonstrates rapid clearance of the Xe-133 from the lungs. Normal half-time for xenon washout is less than 1 minute. Washout is complete within 3 minutes. Retention (trapping) of xenon in the lungs in a focal or in a diffuse pattern is an indication of obstructive lung disease (Fig. 55.4).
![]() Figure 55.4. Chronic Obstructive Pulmonary Disease. A. Ventilation scan, posterior projection, top two rows. Obstructive changes in the middle and upper lobes cause the retention of Xe-133 on a 4-minute washout image (post wo/4). post ib, posterior initial breath; post eq, posterior equilibrium; lpo eq, left posterior oblique equilibrium; rpo eq, right posterior oblique equilibrium; second row, postwashout images 1 to 4 minutes. B. Tc-99m MAA perfusion scan, bottom two rows, labeling is the same as in Figure 55.1. Patchy, inhomogeneous uptake is seen primarily in the middle and upper lung zones. Perfusion defects match those seen on initial breath image of the ventilation scan. |
Normal Tc-99m DTPA aerosol scans resemble normal Tc-99m MAA perfusion scans. However, activity is frequently present within the trachea and the mainstem bronchi, especially in smokers. Swallowed Tc-99m DTPA aerosol is sometimes seen within the esophagus and the stomach.
Normal perfusion scans show well-defined margins of both lungs on all views with sharply defined costophrenic angles. A mild base-to-apex count activity gradient is present due to the physical difference in lung thickness of the base compared with the apex. Tracer distribution should otherwise be homogeneous (Fig. 55.3B).
The heart causes a smoothly defined defect along the left medial lung border that is curvilinear in all projections. A prominent, focal triangular margin suggests the presence of a perfusion defect abutting the heart. The hila are usually seen even in normal patients. Focal asymmetric hilar perfusion defects are abnormal. Cardiomegaly, tortuosity of the aorta, and mediastinal or hilar enlargement cause defects along the medial border of the lung associated with less well-defined corresponding defects on the ventilation scan. The size and shape of any mediastinal structure on the V/Q scan should match its appearance on the CXR.
Abnormal Scans. Focal defects or inhomogeneous tracer distribution is abnormal on either ventilation or perfusion scans. Focal perfusion defects should be compared with the corresponding areas on the ventilation scan and vice versa. The relative size and shape of V/Q defects should then be correlated with the corresponding areas on a recent CXR. Ideally, the correlative CXR should have been performed no more than 6 to 12 hours prior to the V/Q scan since acute findings may change rapidly.
Ventilation scans are abnormal if areas of delayed xenon wash-in or washout are present. Restrictive changes or defects on the single-breath image may disappear on the equilibrium images when xenon bypasses obstructed pulmonary bronchioles through the pores of Kohn and canals of Lambert (see Fig. 55.12). Movement by collateral air drift proceeds more slowly than through the bronchioles, resulting in delayed wash-in and washout. Focal areas of abnormal retention therefore suggest obstructive lung disease (Fig. 55.4, see Fig. 55.7).
Pulmonary Embolism
Pulmonary embolism is one of the common causes of death in the United States. Dahlen and Alpert (5) estimated that 30% of untreated patients with PE die as a consequence of their emboli, in comparison to 10% to 16% mortality for patients treated with anticoagulant therapy. Anticoagulants, however, place patients at significant risk for life-threatening bleeding and should not be prescribed without high probability for the diagnosis of venous thrombosis or PE.
Pulmonary emboli usually originate from thrombi within the deep venous system of the legs and pelvis. Predisposing factors include prolonged immobilization, surgery (particularly intrapelvic or hip surgery), history of prior PE, preexisting cardiac disease, estrogen therapy, smokers, hypercoagulable states such as cancer, and congenital defects of thrombolysis.
PE can be difficult to diagnose clinically. In 70% of patients who survive pulmonary emboli, the emboli may not be clinically suspected (6). The classic triad of dyspnea, hemoptysis, and pleuritic chest pain occurs in less than 20% of patients with pulmonary emboli. Larger emboli increase the likelihood of symptoms (7,8). Symptoms associated with PE, however, are nonspecific. Pulmonary infection or inflammation, pneumothorax, cancer, and cardiac disease may produce similar symptoms. An electrocardiogram should be performed in patients suspected of having PE to detect cardiac causes for chest pain or dyspnea. If a patient develops acute cor pulmonale because of pulmonary emboli, the electrocardiogram will show signs of right heart strain.
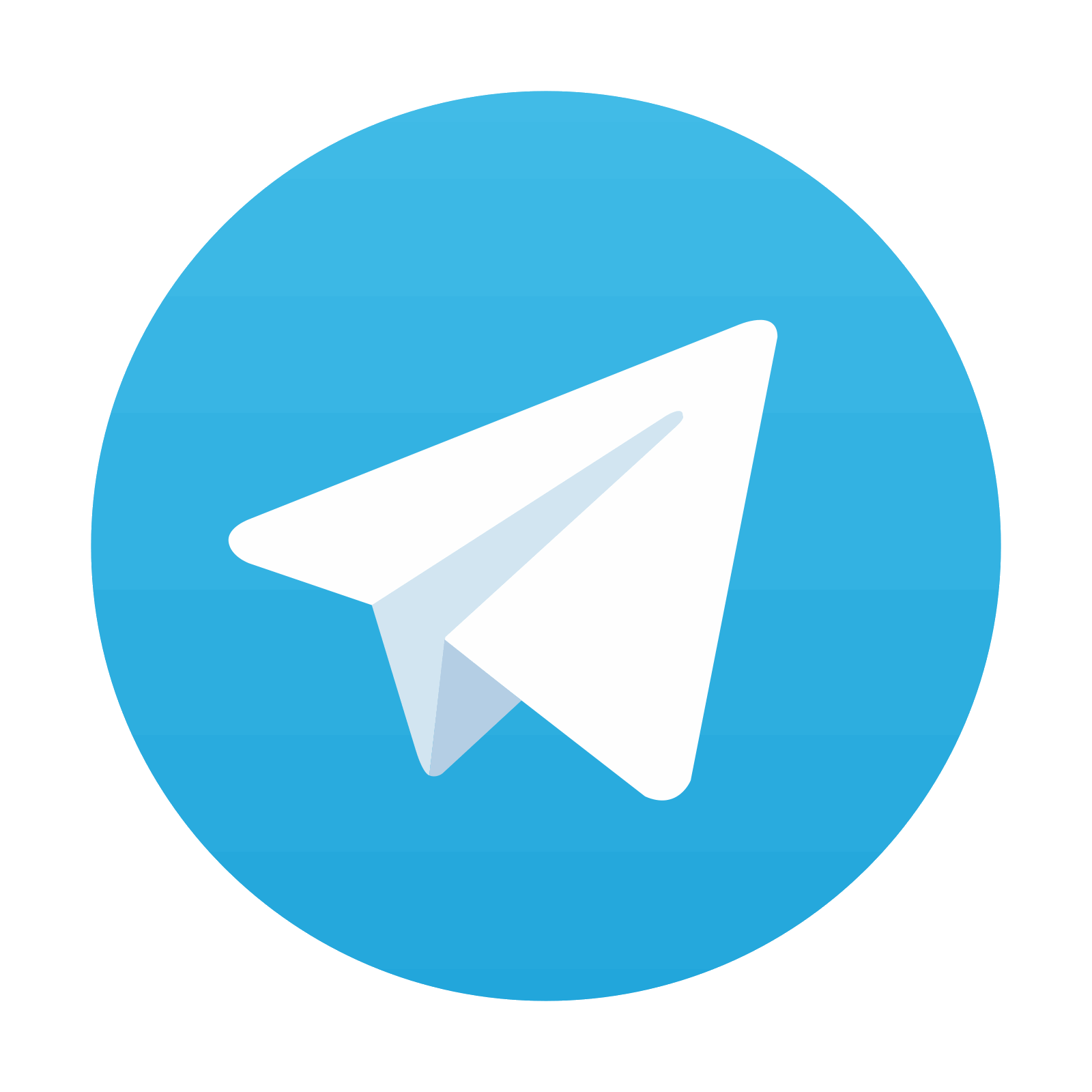
Stay updated, free articles. Join our Telegram channel
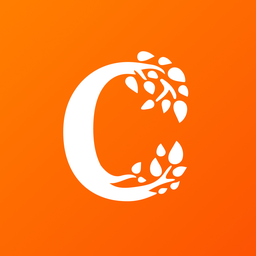
Full access? Get Clinical Tree
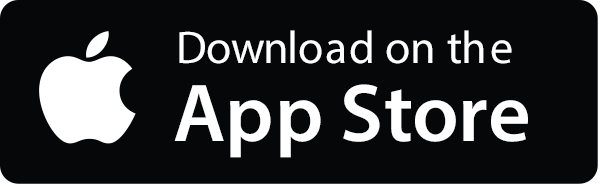
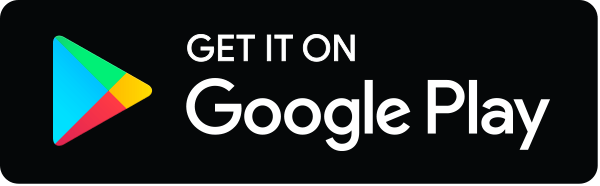