Radiation therapy is an important modality in the management of patients with intrathoracic malignant neoplasms and primary and secondary chest wall neoplasms. Radiation therapy for these tumors usually results in radiation of the adjacent lung, and radiation-induced lung injury has been reported in association with treatment of lung, breast, esophageal, and thymic neoplasms and in lymphoma and pleural mesothelioma. Adverse effects of radiation therapy on the lung parenchyma—in particular, acute pneumonitis and chronic fibrosis—include ground-glass opacities or consolidation in the acute phase and traction bronchiectasis, volume loss, architectural distortion, and consolidation in the late phase.
Improvements in radiation techniques and delivery, including three-dimensional (3D) conformal radiotherapy (3D-CRT), intensity-modulated radiotherapy (IMRT), stereotactic body radiotherapy (SBRT), proton therapy, and altered fractionation schedules, have improved locoregional control and survival in patients with non–small cell lung cancer (NSCLC). Of importance, the imaging manifestations of radiation-induced lung disease associated with these newer therapeutic approaches often differ from those seen with conventional radiation therapy.
Knowledge of the expanded spectrum of manifestations of radiation-induced lung disease is important in the management of patients after radiation therapy and is useful in the diagnosis of local recurrence of malignant disease and in differentiating radiation-induced lung disease from infection. In this chapter we review the etiology and clinical and imaging manifestations of radiation-induced lung disease and emphasize unexpected or atypical manifestations that can occur when newer radiation techniques are used.
Etiology
Radiation-induced lung disease rarely occurs with fractionated total doses less than 20 Gy and is commonly present in patients who receive doses greater than 60 Gy. A pooled analysis of 24 studies of 1911 patients with both small cell and NSCLC who received chemoradiation showed that the incidence of clinically significant radiation-induced pneumonitis (grade 2–3 according to the Radiation Therapy Oncology Group/European Organization for Research and Treatment of Cancer criteria) was 6% for total doses less than 45 Gy and 12% for doses greater than 55 Gy. Although the total dose of radiation delivered is important in the development of radiation-induced lung disease, other radiation technique factors that affect lung injury are the fractions into which the total dose is divided, the dose rate, and the volume of lung irradiated. The injury sustained by the lungs tends to decrease as the number of fractions in which the radiation is delivered increases.
Improved techniques of radiation therapy and dose escalation can modify the development and extent of lung injury. For instance, because the new fractionation strategies are often associated with an increase in radiation dose, there is a tendency to increase acute toxicity. However, by decreasing the treated volume, aggressive hyperfractionated schedules can limit acute radiation-induced injury of adjacent lung.
Various patient factors can increase the degree of injury sustained by the lung after irradiation of a thoracic neoplasm; these include age, low performance status, cigarette smoking, preexisting lung disease, and prior radiation therapy. The effect of neoadjuvant or concurrent chemotherapy on radiation-induced lung injury is not clear; some studies report an increase, whereas others have reported no significant association with pneumonitis. In addition, although steroids ameliorate radiation pneumonitis, an abrupt termination of administration can unmask latent radiation injury to the lung. Conversely, cytoprotectants such as amifostine, an organic thiophosphate, have shown promise in reducing the incidence and severity of injury associated with radiation therapy, although they have not been established as standard of care in preventing pulmonary toxicity.
Prevalence
Because of the complexity of factors that determine whether a patient with a thoracic malignant neoplasm will receive radiation therapy, including histology, stage, symptoms, and performance status, together with the heterogeneity of treatment options and numerous factors that can influence the degree of injury sustained by the lung after radiation therapy, the true prevalence of radiation-induced lung disease is difficult to ascertain for patients with lymphoma; pleural mesothelioma; and lung, esophageal, breast, and thymic cancers. However, radiation-induced lung injury is common in these patients, and subclinical changes in pulmonary function test results occur in an even larger percentage of patients.
Of the multiple thoracic malignancies, patients with lung cancer often receive the highest doses of radiation to the largest volume of lung and are accordingly more likely to develop radiation-induced lung disease. On the basis of a reported incidence of 10% to 20%, moderate to severe radiation-induced pneumonitis will probably occur in approximately 15,000 to 25,000 patients with lung cancer in 2016. This incidence is likely underestimated because the nonspecific symptoms of radiation-induced lung disease can be misattributed to preexisting respiratory or cardiovascular disease.
Radiation Therapy Planning and Treatment Terminology
The goal of radiation therapy planning is to deliver a high dose of radiation to the tumor while sparing the surrounding normal tissue. To enable systematic tumor volume delineation, the International Commission on Radiation Units and Measurements has specified the use of concentric target volume delineations before radiation therapy. The gross tumor volume (GTV) is obtained outlining visible malignancy, including any involved nodes, on imaging studies. Next, a margin around the GTV is added for potential microscopic subclinical disease and is called the clinical target volume. An additional margin is added to the treatment plan to account for possible tumor movement during treatment because of respiratory motion and is called the internal target volume. The final volume treatment plan is the planning target volume and accounts for anticipated differences in patient positioning during the delivery of treatment.
Types of Radiation Therapy Delivery
Sophisticated radiation therapy techniques, such as 3D-CRT, IMRT, and SBRT, allow the delivery of high therapeutic dose to the tumor, decreased radiation dose to adjacent tissue, and improved local tumor control rate. In contrast to conventional radiation therapy treatment planning, 3D-CRT uses a 3D image reconstructed from computed tomography (CT) scanning data to determine the target volumes to be treated. A computer planning system is used to design beam arrangements with a variety of orientations that deliver maximal radiation dose to the tumor while limiting exposure to normal structures. In IMRT the combination of powerful computer planning algorithms with multileaf collimators results in a high-precision dose delivered to the tumor with a steep dose fall-off outside the target, which decreases the volume of normal tissue that is radiated. Murshed and colleagues, in a study of 41 patients with advanced-stage NSCLC (stage III–IV), demonstrated a 10% improved target conformity of IMRT when compared with 3D-CRT. SBRT has steep dose gradients, allowing delivery of a highly precise dose conformed to the shape of the tumor. SBRT uses only a few fractions of radiation while delivering a significantly high biologic effective dose (>100 Gy). Because the target volume is small, SBRT is being used more frequently with curative intent in patients with peripheral early-stage NSCLC ( Fig. 66.1 ). In these patients SBRT is used rather than conventional radiation therapy or surgical resection as it achieves local control rates of 80% to 95% and improves overall survival compared with conventional radiation therapy.
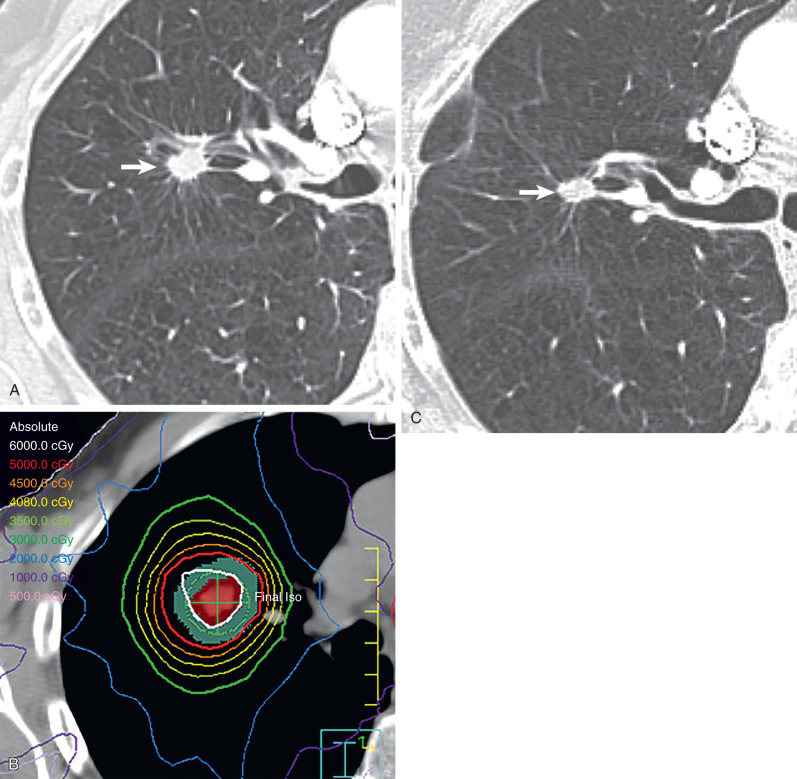
Radiation Therapy Planning and Treatment Terminology
The goal of radiation therapy planning is to deliver a high dose of radiation to the tumor while sparing the surrounding normal tissue. To enable systematic tumor volume delineation, the International Commission on Radiation Units and Measurements has specified the use of concentric target volume delineations before radiation therapy. The gross tumor volume (GTV) is obtained outlining visible malignancy, including any involved nodes, on imaging studies. Next, a margin around the GTV is added for potential microscopic subclinical disease and is called the clinical target volume. An additional margin is added to the treatment plan to account for possible tumor movement during treatment because of respiratory motion and is called the internal target volume. The final volume treatment plan is the planning target volume and accounts for anticipated differences in patient positioning during the delivery of treatment.
Types of Radiation Therapy Delivery
Sophisticated radiation therapy techniques, such as 3D-CRT, IMRT, and SBRT, allow the delivery of high therapeutic dose to the tumor, decreased radiation dose to adjacent tissue, and improved local tumor control rate. In contrast to conventional radiation therapy treatment planning, 3D-CRT uses a 3D image reconstructed from computed tomography (CT) scanning data to determine the target volumes to be treated. A computer planning system is used to design beam arrangements with a variety of orientations that deliver maximal radiation dose to the tumor while limiting exposure to normal structures. In IMRT the combination of powerful computer planning algorithms with multileaf collimators results in a high-precision dose delivered to the tumor with a steep dose fall-off outside the target, which decreases the volume of normal tissue that is radiated. Murshed and colleagues, in a study of 41 patients with advanced-stage NSCLC (stage III–IV), demonstrated a 10% improved target conformity of IMRT when compared with 3D-CRT. SBRT has steep dose gradients, allowing delivery of a highly precise dose conformed to the shape of the tumor. SBRT uses only a few fractions of radiation while delivering a significantly high biologic effective dose (>100 Gy). Because the target volume is small, SBRT is being used more frequently with curative intent in patients with peripheral early-stage NSCLC ( Fig. 66.1 ). In these patients SBRT is used rather than conventional radiation therapy or surgical resection as it achieves local control rates of 80% to 95% and improves overall survival compared with conventional radiation therapy.
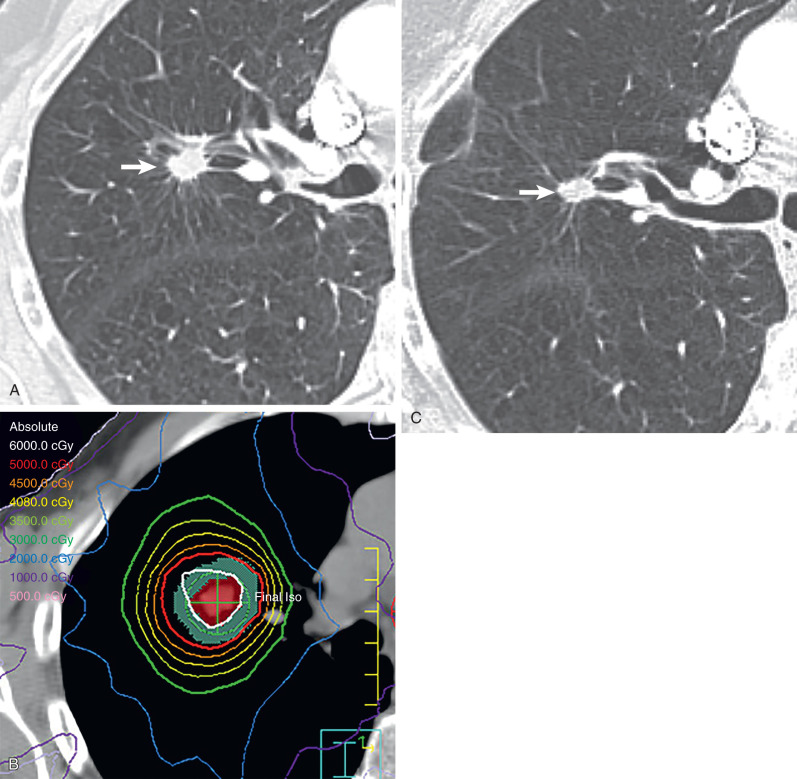
Recent Innovations in Radiation Therapy
Radiation therapy has improved considerably during the past few decades by refinements of existing techniques and introduction of new technology, including positron emission tomography (PET)-CT using the radiopharmaceutical 18 F-deoxyglucose, a d -glucose analog labeled with fluorine-18 ( 18 F-FDG–PET-CT), four-dimensional (4D) treatment planning, and proton therapy.
The increasing use of advanced CRT techniques such as IMRT and SBRT in thoracic radiation oncology makes precise target delineation important as these techniques have a higher likelihood of geographic misses. Use of FDG–PET-CT and 4D treatment planning improves the precision in radiation therapy by increasing the accuracy of tumor delineation and minimizing damage to surrounding structures.
FDG–PET-CT improves delineation of the extent of the tumor compared with CT alone when there is associated postobstructive atelectasis. The use of FDG–PET-CT results in less variation in lung cancer contouring compared with CT alone and reduces the risk of geographic misses with SBRT. In addition, because tumor volume delineation by FDG–PET-CT is typically smaller than that defined by CT, this can potentially facilitate dose escalation without increasing side effects. Also, the detection of unsuspected nodal metastases by FDG–PET-CT improves the target accuracy for nodal radiation and decreases isolated nodal failures. In addition to assisting with target volume delineation, PET-CT imaging may have an important role in adaptive planning. Adaptive planning is the modification of a radiation treatment plan during the course of therapy to account for changes in the tumor and/or adjacent tissue. The use of FDG–PET-CT has been reported to allow a smaller target volume to be radiated during radiotherapy delivery and to facilitate dose escalation.
The use of 4D imaging in radiation treatment planning introduces the concept of time to the 3D conformal treatment planning and compensates for tumor motion, patient movement, and movement occurring either during a single fraction (intrafraction) or between multiple fractions (interfraction) of radiation. Intrafractional movement can cause significant target deformation resulting from respiratory motion, and interfractional variation can interfere in tumor delineation as tumor and anatomy may change significantly between each treatment session. 4D treatment planning allows an assessment of tumor motion across an entire respiratory cycle, and radiation therapy is delivered accordingly. 4D-CT is currently being used in conjunction with 3D-CRT and IMRT to achieve high-precision radiation dose delivery to the tumor. In addition, in the treatment of lung cancer, using SBRT with 4D-CT imaging allows a significant reduction of target volumes and decreased dose to surrounding tissue when compared with conventional 3D-CT. 4D-CT–based planning has also optimized radiation delivery in patients with lung cancer receiving proton therapy. In patients with target volumes that have a large amount of motion (typically >1 cm), two approaches can be used to further reduce the amount of lung treated. The first, respiratory gating, allows delivery of radiation only during certain phases of the respiratory cycle while the patient breathes normally. The second, deep inspiratory breath hold , has the patient “hold his or her breath” after deep inspiration. Because radiation is delivered when the tumor is in a relatively “fixed” position with either of these approaches, the full extent of the tumor motion does not need to be delineated.
Proton therapy can allow high-dose radiation delivery to the tumor with minimal radiation exposure to the adjacent organs. The advantage of proton therapy relies on the physical characteristics of the proton particles, which deposit nearly all the therapeutic dose at a particular depth (Bragg peak), and this typically results in a negligible exit dose beyond the target. However, in aerated lung, proton beams travel beyond the distal edge of the tumor and can cause damage to adjacent lung tissue. A study assessing dosimetric values for stage I and III NSCLC treated with proton therapy, compared with 3D-CRT and IMRT, has shown that all three techniques provide adequate tumor coverage, but proton therapy delivers lower doses to the adjacent lung, esophagus, and spinal cord than the two other techniques. Sejpal and colleagues compared photon and proton-based RT and showed that proton therapy resulted in lower rates of esophagitis and severe pneumonitis than 3D-CRT and IMRT. However, the use of proton therapy in patients with lung cancer is not clearly defined. Currently, proton therapy has been indicated for centrally located tumors adjacent to the heart, spinal cord, and esophagus ( Fig. 66.2 ) as well as tumors close to the brachial plexus. In addition, a recent phase II randomized study from The University of Texas M.D. Anderson Cancer Center highlighted the need for adequate patient selection for this approach. Patients with locally advanced NSCLC were randomized to IMRT or passive scattering proton therapy (proton therapy using 3D-conformal techniques). The coprimary end points were the incidence of radiation pneumonitis and locoregional control, and in this unselected patient population, there was no difference between the two modalities. Further analyses are underway comparing IMRT with intensity-modulated proton therapy (proton therapy incorporating features of inverse planning and with greater conformality than the passive scattering approach), which use selective dose escalation based on normal tissue doses.
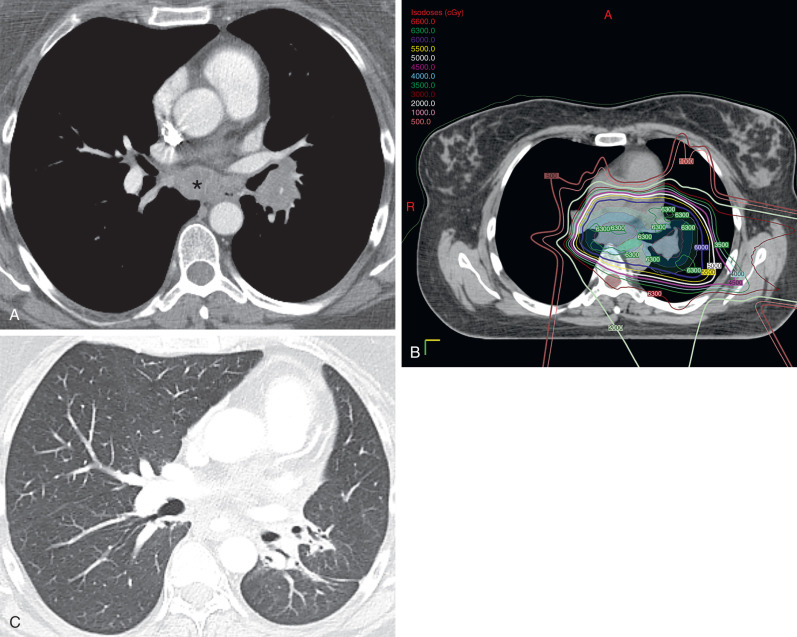
Dose and Fractionation
External-beam radiation therapy is an established treatment option for patients with nonresectable locally advanced (stage III) NSCLC. Typically, a standard dose of 60 Gy, given in a single fraction per day, 5 days a week, during 6 weeks is used. The use of this dose has been recently confirmed in a comparison study as 74 Gy in a phase III national trial. However, because changing the time interval and the dose of radiation can influence the response of both normal and tumor tissues to radiotherapy and can optimize radiation treatment, different fractionation schedules have also been assessed as treatment options for stage I to III NSCLC. In the hyperfractionation scheme, there is delivery of more than one fraction in 24 hours, using a larger number of fractions per week (between 10 and 25 fractions) and using a dose per fraction of less than 1.8 Gy. Although several hyperfractionation regimens have been tested in randomized trials for NSCLC, it has not been shown to be superior to standard fractionated regimens. Currently, a hyperfractionated schedule is selectively used for patients with NSCLC located near the spinal cord and brachial plexus, as late side effects can occur after high doses with a standard fractionation regimen. However, in the setting of small cell lung cancer, a hyperfractionated regimen of 1.5 Gy delivered twice daily to 45 Gy is one of a limited number of acceptable treatment options and is currently being further assessed in a phase III randomized trial.
In addition, accelerated fractionation can be used. Accelerated fractionation is related to the intensity of the treatment over time in which the rate of dose accumulation is greater than 10 Gy per week. One type of accelerated radiotherapy is called continuous hyperfractionated accelerated radiotherapy (CHART), which combines hyperfractionation and accelerated schemes in a 54-Gy treatment, given in three fractions a day, 1.5 Gy per fraction, during 12 days. Saunders and colleagues compared the CHART scheme with standard radiation therapy and demonstrated improved survival in NSCLC patients.
An alternative to hyperfractionation is hypofractionation, which includes a larger dose per fraction (>3.0 Gy) and fewer fractions, 1 to 4 days a week, and is the basis of SBRT.
Clinical Presentation
The description of classic radiation-induced lung injury has three main phases: (1) an initial latent phase (first 3–4 weeks after completion of therapy); (2) an early phase: acute exudative pneumonitis (3 weeks to 6 months); and (3) a late phase: pulmonary fibrosis (6 months and later). Radiation-induced lung disease usually manifests clinically as acute pneumonitis 4 to 12 weeks after completion of radiation therapy, although symptoms occasionally occur as early as 1 month after the beginning of radiation therapy and as late as 6 months after completion of treatment. The earlier clinical presentation of acute pneumonitis can occur with the newer treatment regimens that use hyperfractionated accelerated radiation. For instance, in a study evaluating escalated hyperfractionated accelerated radiation therapy in locally advanced NSCLC, acute pneumonitis developed in 40% of the patients, and onset was typically before the completion of treatment (median, 4 weeks after beginning of treatment; range, 3–5 weeks).
In general, clinical symptoms are proportional to the extent of the radiation-induced lung injury and pretreatment pulmonary function of the patient. Symptomatic patients typically present with cough and mild dyspnea, although patients can have severe respiratory compromise. In addition, patients can occasionally present with chest pain, fever associated with nonproductive cough, or cough productive of small amounts of blood-tinged sputum.
Acute radiation-induced pneumonitis manifesting as mild to moderate dyspnea generally resolves with treatment. In the 6.7% to 16% of patients with NSCLC who develop severe respiratory distress, morbidity and mortality can be high. In fact, Wang and colleagues reported that severe acute radiation-induced pneumonitis was associated with a mortality rate approaching 50% in the first 2 months after the onset of symptoms. Also, although IMRT after extrapleural pneumonectomy in patients with pleural mesothelioma has not typically been associated with radiation-induced injury in the remaining lung, 6 of 13 patients (46%) in a study developed severe pneumonitis in the contralateral lung 5 to 57 days (mean, 30 days) after completion of therapy, with subsequent demise of all 6 patients.
It is important to be aware that (1) symptomatic radiation-induced pneumonitis does not predict subsequent fibrosis, and (2) the clinical manifestations of acute radiation-induced pneumonitis often cannot be accounted for by the radiation therapy received by the patient (i.e., the dyspnea is more severe than would be expected from the volume of lung irradiated). It is postulated that a hypersensitivity reaction occurs in response to localized lung irradiation and results in an out-of-field pneumonitis.
In the late phase of radiation-induced lung injury, pulmonary fibrosis localized to the region of the irradiated lung typically develops after 6 months. Most patients with radiation fibrosis are asymptomatic, although dyspnea that varies from minimal to severe can be present. Symptoms are usually minimal if fibrosis is limited to less than 50% of one lung. Chronic respiratory failure and cor pulmonale secondary to fibrosis of a large volume of lung can occasionally occur as late manifestations of radiation-induced lung disease.
Pathophysiology
Radiation-induced lung injury has classically been described as separate phases encompassing acute pneumonitis and fibrosis that occur at different times after radiation therapy. However, radiation-induced lung injury can be viewed as a continuum, with no distinct separation between the temporal sequences of the different phases of injury. In terms of the pathophysiologic process, radiation therapy initiates a sequence of molecular and genetic changes resulting in lung injury. The biologic effects of ionizing radiation begin with the overproduction of oxygen free radicals, more generally known as reactive oxygen species. Reactive oxygen species result in damage to endothelial cells and exudation of proteinaceous material into the alveoli, desquamation of epithelial cells from the alveolar wall, and infiltration of inflammatory cells. Depending on the severity of injury, these pathologic exudative changes may resolve in a few weeks to months or result in an acute radiation pneumonitis.
The continuous overproduction of reactive oxygen species after the initial injury may occur for months or years after the completion of radiation therapy and result in the progressive tissue damage, loss of parenchymal cells, and fibrosis that are histologically typical of late radiation lung injury. Uncommonly, radiation-induced lung injury is manifested as organizing pneumonia, most commonly outside the expected radiation field, and chronic eosinophilic pneumonia, particularly in patients with history of asthma or atopy.
Lung Function
The severity of pneumonitis (grade 1–5) is usually defined clinically by the Southwest Oncology Group standard response criteria or the toxicity criteria of the Radiation Therapy Oncology Group and the European Organization for Research and Treatment of Cancer, according to the absence or presence of dyspnea and cough and the treatment required by the patient. However, unfortunately, these grading systems are to a large extent subjective and difficult to correlate with pulmonary function test results. Fifty percent to 90% of patients experience a decline in pulmonary function test results after radiation therapy, and these tests have been comprehensively evaluated in an effort to predict the incidence and severity of radiation-induced pneumonitis. Pulmonary function test results are relatively normal in most patients in the 4 to 8 weeks after completion of radiation therapy. Subsequently, a wide range of changes can occur in pulmonary function in both the acute pneumonitis phase and late fibrotic phase. Decreases have been noted in vital capacity, inspiratory capacity, total lung capacity, residual volume, forced expiratory volume in 1 second, and carbon monoxide diffusing capacity (DLCO). DLCO is generally the most severely affected parameter, and the impairment can progress with a reported annual decrease of 3.5% after the first year that follows radiation therapy completion.
Manifestations of the Disease
General
The histopathologic response of the lungs to radiation injury is limited and manifested radiologically as two major distinct patterns. To describe these patterns of radiation-induced lung disease, the end of radiation therapy has traditionally been used as a reference point for the temporal sequence of changes because the length of treatment varies, and thus reference from the beginning of treatment would be inconsistent. Typically, acute radiation pneumonitis occurs 4 to 12 weeks after completion of radiation therapy and is followed within 6 to 12 months by radiation-induced fibrosis in the region of the radiation-induced lung injury.
The criteria for the diagnosis of radiation-induced organizing pneumonia include radiotherapy within 12 months, symptom duration of at least 2 weeks, pulmonary airspace opacities outside the radiation treatment field on chest radiography or CT, and exclusion of other causes. Diagnostic criteria for radiation-induced chronic eosinophilic pneumonia are similar to those associated with organizing pneumonia but include the presence of blood eosinophilia higher than 1 × 10 9 /L or more than 40% eosinophils in the differential cell count of bronchoalveolar lavage fluid.
Radiography
The acute phase of radiation-induced lung disease initially is manifested radiographically as ground-glass opacities or consolidation in the treatment field ( Fig. 66.3 ). Although radiation pneumonitis usually occurs within the irradiated lung, radiation pneumonitis outside the treatment fields has been reported. Ipsilateral pleural effusions can occur after radiation therapy and usually develop at the time of radiation pneumonitis (i.e., within 6 months after completion of therapy). Pleural effusions that develop after 6 months, continue to increase in size, or present as large effusions may require thoracentesis for differentiation of benign from malignant disease.
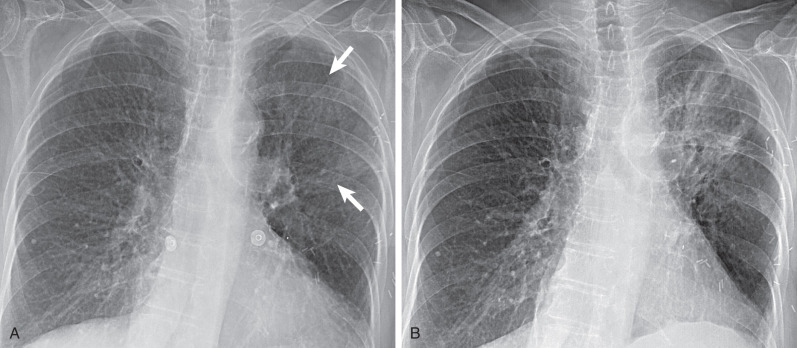
Although the opacities of radiation pneumonitis can gradually resolve without radiologic sequelae when the injury to the lung is limited, in cases of more severe injury, there is usually a progression to fibrosis. The majority of fibrosis will occur within the first 12 months, and the development commonly progresses slowly in the 6 to 12 months after completion of therapy and stabilizes within 2 years. Radiation fibrosis is manifested radiologically as a well-defined area of volume loss, linear scarring, consolidation, and traction bronchiectasis. Consolidation usually coalesces and typically has a relatively sharp margin that conforms to the treatment field rather than to anatomic boundaries ( Fig. 66.4 ). With the evolution of radiation fibrosis, the demarcation between normal and irradiated lung parenchyma often becomes more sharply defined.
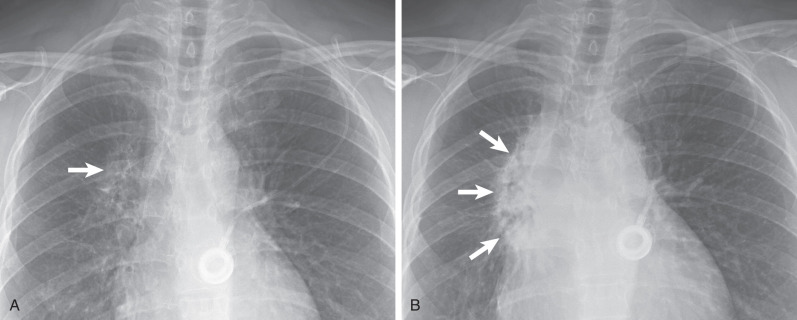
Computed Tomography
CT is more sensitive than chest radiography in detecting radiation-induced lung disease. Ground-glass opacities representing early radiation pneumonitis ( Fig. 66.5 ) can be seen a few weeks after completion of radiation therapy, although the radiograph is often normal. With some of the newer treatment regimens, opacities can sometimes be observed on CT before or at completion of radiation therapy. On occasion, acute pneumonitis detected on CT can appear nodular and simulate metastatic nodules. Typically, the nodules of radiation pneumonitis will be within the lung that underwent radiation therapy and are irregular or poorly marginated ( Fig. 66.6 ). The nodules usually coalesce into areas of consolidation and eventually become a component of the radiation fibrosis. CT is the optimal modality for assessment of the evolution and chronic manifestations of radiation-induced lung injury ( Fig. 66.7 ) and for the detection of locoregional recurrence of malignant disease (see the section on differential diagnosis).
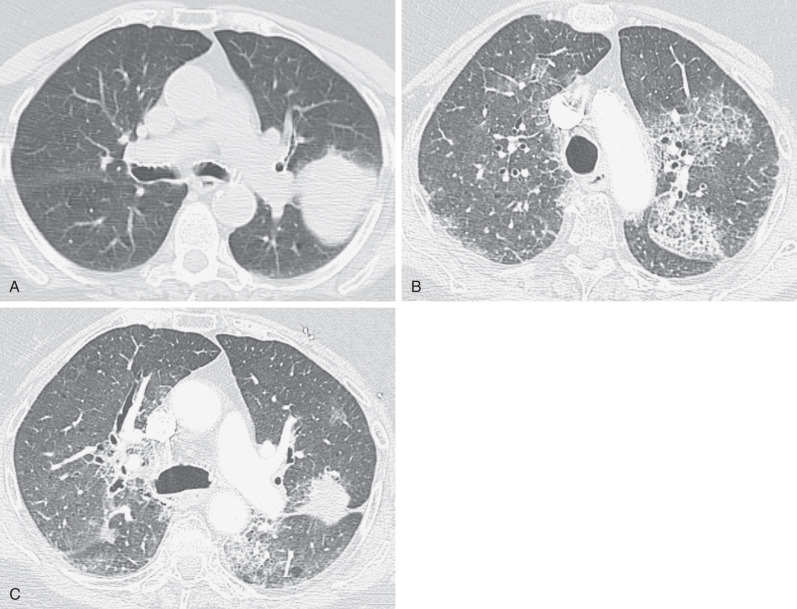
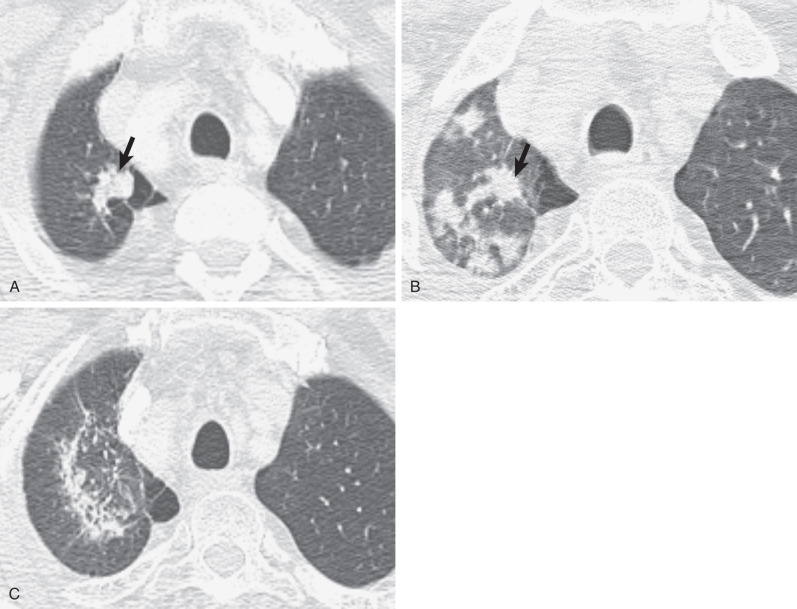
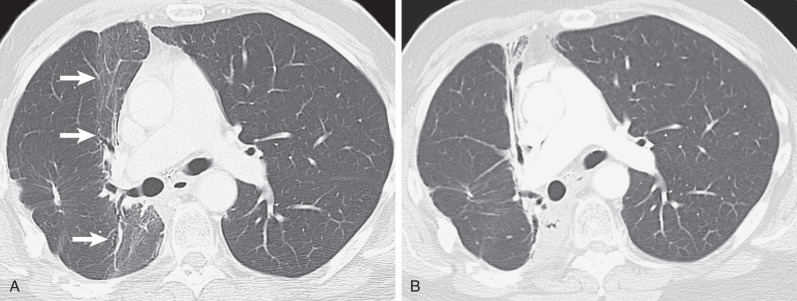
The technique used to deliver radiation will affect the CT manifestations of radiation-induced lung disease. Radiation-induced lung disease varies in shape and distribution with new radiation delivery techniques as the radiation dose is altered according to the location, extent, and type of malignant neoplasm for delivery of a tumoricidal dose while limiting the dose of radiation to adjacent lung. Recognition of imaging manifestations of this treatment can be facilitated with computed dosimetric reconstruction, whereby treatment volumes are superimposed on CT images ( Figs. 66.8 and 66.9 ). In addition, the use of the newer methods of delivering radiation can result in lung opacities at unusual locations away from the site of disease that can be mistaken for other disease entities. For instance, when an NSCLC is treated, radiation-induced lung disease can manifest as modified conventional (consolidation, volume loss, and bronchiectasis similar to but less extensive than conventional radiation fibrosis), scar-like (linear opacity in the region of the original tumor ( Fig. 66.10 ), and mass-like patterns ( Fig. 66.11 ). Although diagnostic confusion is unlikely to arise from the modified conventional and scar-like patterns, the mass-like pattern can easily be misinterpreted as malignant neoplasia in the absence of a history of new methods of radiation therapy delivery. In accordance, it is important to be aware that the newer techniques of 3D-CRT, IMRT, SBRT, and proton therapy often distribute dose to unexpected areas, and to avoid diagnostic confusion, the specific isodose patterns associated with each individual patient plan need to be recognized.
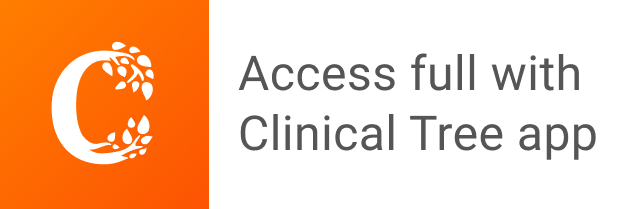