The anatomic and functional characteristics of the aorta, which may at first glance appear relatively straightforward, are now recognized to be complex. Recent insights from both modern imaging technology and better understanding of the hydraulic principles associated with the variety of aortic diseases have helped the medical community to realize the multiple facets of in vivo aortic pathology, as well as its varied clinical presentation.
Diagnostic modalities such as transesophageal echocardiography (TEE), cardiovascular magnetic resonance (CMR), and spiral computed tomography (CT) have all been shown to be useful to interrogate the aorta, both in chronic disease and in acute aortic syndromes. X-ray contrast angiography, the former gold standard in acute and chronic aortic syndromes, has been relegated to a secondary role after the emergence of the noninvasive techniques, most importantly CMR, with their high sensitivity, specificity, and practical advantages. However, none of the diagnostic modalities listed above is ideal for all patients, and for a given individual, knowledge of both accuracy and limitations in the presenting clinical scenario are required. Although the information content of CMR may greatly overlap with established methods such as echocardiography, CT, or angiography, the technique is more comprehensive and offers more options including four-dimensional (4D) functional imaging.
Besides images with high soft tissue contrast without any radiation, CMR can demonstrate and quantify functional parameters beyond anatomic depiction. Combining anatomical and functional information in a single acquisition means that CMR can potentially provide a more comprehensive evaluation of thoracic aortic disease, including aortic valve morphology and function. CMR is an ideal imaging modality for surveillance in a relatively young patient population requiring long-term or even lifelong follow-up care. Although the cost-effectiveness of CMR has not been proven in all areas, CMR is the preferred modality in both aortic disease, including aneurysm and dissection, and its precursors, and in congenital and inherited heart diseases. This chapter focuses on emerging advantages of CMR with respect to a spectrum of aortic pathologies.
Principles of Cardiovascular Magnetic Resonance in Aortic Imaging
Spin Echo Cardiovascular Magnetic Resonance
Spin echo T1-weighted imaging provides the anatomic detail of the aortic wall and pathologic conditions such as atheromatous plaques, intimal flaps, or intramural hemorrhage and is still the basis of any aortic study, whereas T2-weighted images (repetition time: 2/3 RR; echo time: 80 to 100 ms) can be used in tissue characterization of the aortic wall or blood components. Electrocardiogram (ECG) triggering is essential in minimizing motion and pulsatility artifacts. Slice thickness of 3 to 8 mm and an echo time (TE) of 20 to 30 ms are standard, whereas repetition time (TR) is determined from the RR interval of the ECG. A shorter acquisition time can be achieved with fast spin echo pulse sequences whereby a long train of echoes is acquired by using a series of 180-degree radiofrequency (RF) pulses; washout effects are even more substantial than in conventional spin echo techniques. A superior black-blood effect is achieved by using preparatory pulses (such as presaturation, dephasing gradients, and preinversion) with one or more additional RF pulses outside the plane to suppress the signal intensity of in-flowing blood and nullify the blood signal ( Fig. 43.1 ). Therefore “black-blood” fast T1-weighted and T2-weighted spin echo sequences have improved image quality, and constitute the method of choice for morphologic assessment of the thoracic aorta. Images are acquired in axial and additional planes, depending on the anatomy and diagnostic problems, to define the extent of the disease in three-dimensional (3D) space.
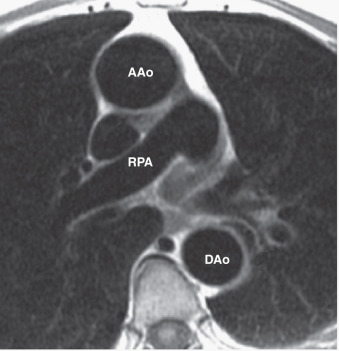
Gradient Echo Cardiovascular Magnetic Resonance and Flow Mapping
Gradient echo techniques provide dynamic and functional information, although with fewer details of the vessel wall. The bright signal of the blood pool on gradient echo images results from flow-related enhancement obtained by applying RF pulses to saturate a volume of tissue. With a short TR (4 to 8 ms) and low flip angle (20 to 30 degrees), maximal signal is emitted by blood flowing in the voxel and ECG-gated acquisition provides a high degree of temporal resolution throughout the cardiac cycle (up to 20 to 25 frames) to be displayed in cine format. Flow-related enhancement is produced by inflow of unsaturated blood exposed to only one RF pulse. As result, the laminar moving blood displays a bright signal in contrast to stationary tissues. The signal can be reduced if the flow is low, as in aortic aneurysms. Mural thrombi can be identified by persistent low-signal intensity in different phases of the cardiac cycle. Turbulent flow produces rapid spin dephasing and results in a signal void, providing additional information in many pathologic conditions such as coarctation, aortic valve insufficiency, aortic aneurysm, and dissection. Particularly in aortic dissection, the detection of entry and reentry sites is a special capability of functional CMR that can be helpful in planning both surgical and endovascular therapy. Accurate quantitative information on blood flow is obtained from modified gradient echo sequences with parameter reconstruction from the phase rather than the amplitude of the magnetic resonance (MR) signal; this is also known as flow mapping or phase contrast or velocity-encoded cine CMR ( Fig. 43.2 ). In each pixel of velocity images, the phase of the signal is related to the velocity component in the direction of a bipolar velocity phase-encoding gradient. In the phase image, the velocity of blood flow can be determined for any site of the vascular system. Flow velocity is calculated by using a formula in which velocity is proportional to change in the phase angle of protons in motion. MR maps of flow velocity are obtained two-dimensionally, which is particularly important in profiles of nonuniform flow, such as in the great vessels. Quantitative data on flow velocity and flow volume are obtained from the velocity maps through a region of interest. The mean blood flow is estimated by multiplying the spatial mean velocity and the cross-sectional area of the vessel. Vector mapping has been used to describe flow patterns in different aortic diseases (e.g., hypertension, aneurysms, dissection, Marfan syndrome, coarctation).
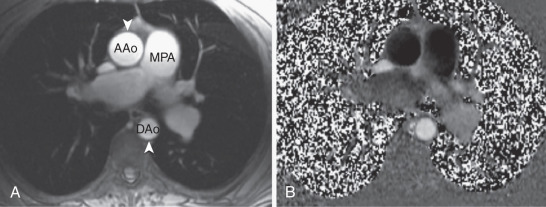
Magnetic Resonance Angiography
A variety of magnetic resonance angiography (MRA) techniques, including various pulse sequences, methods of data acquisition, and postprocessing, have been developed, but first-pass 3D contrast-enhanced MRA constitutes the method of choice for the evaluation of the aorta. The technique relies on the contrast-induced T1-shortening effects of the gadolinium (Gd) contrast agent, whereby saturation problems with slow flow or turbulence-induced signal voids are avoided. During the short intravascular phase, the paramagnetic contrast agent provides a precise signal in the arterial or venous system, enhancing the vessel-to-background contrast-to-noise ratio, irrespective of flow patterns and velocity and not only delineates the dissection lamella, but also filling of critical side branches. Intravenous bolus timing is necessary to ensure peak enhancement during the middle of CMR acquisition and not exceeding the acquisition time. Improved gradient systems allow a considerable reduction of the minimum TRs and TEs and the acquisition of complex 3D datasets within a breath-hold interval of under 30 seconds. With the support of maximum intensity projection (MIP) images and the 3D multiplanar reformation, this technique delineates all the morphologic details of the aorta and its side branches in any plane in a 3D format ( Fig. 43.3 ). Some caution has to be exercised in patients with poor renal function because this agent has been associated with the development of nephrogenic systemic fibrosis. From a technical point of view, blood pool imaging can be acquired without the use of a contrast agent using an ECG-gated and respiratory-navigated balanced steady-state free precession (SSFP) acquisition. After an intervention, first-pass contrast-enhanced angiography is instrumental in assessing the success of a procedure and in quantifying false lumen thrombosis, a well-established prognostic indicator. The image acquisition is timed according to the arrival of the contrast bolus in the proximal unaffected aorta and thrombosis is assumed to be present when there is no contrast agent in the false lumen. However, recent studies have shown that the flow rates in the false lumen are highly variable and often very slow. The use of a new CMR technique with gadofosveset trisodium blood pool agent in equilibrium state is encouraging.
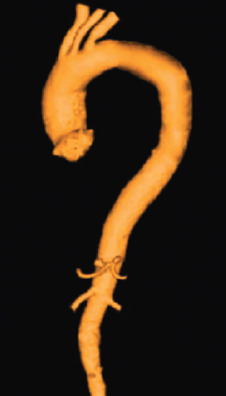
Finally, phase contrast sequences may be used to assess blood flow and velocity in both lumens of a dissection. This imaging technique does not play a major role in the context of diagnostic imaging; however, it appears to be an interesting research tool and potentially provides parameters for a prognostic assessment of a given patient. These sequences can also be used to acquire 3D velocity information (Vx, Vy, Vz) for each voxel, within a 3D volume, over time (frequently called 4D phase contrast-CMR) ( Fig. 43.4 ). This acquisition offers the potential to study aortic hemodynamics, flow patterns, and derived vessel wall parameters, such as wall shear stress. This technique can help to identify entry tears between the true and false lumens and to stratify patients according to their risk of aneurysm formation.
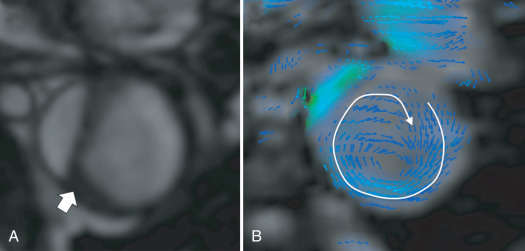
Four-dimensional time-resolved angiography with keyhole (TRAK) is a contrast-enhanced MR angiography technique that can acquire multiple time-resolved 3D volumes over time using image acceleration techniques. It can be used to acquire several phases of contrast distribution, including, but not limited to, the arterial and venous phases. This technique can be used clinically to characterize flow-related phenomena, such as false lumen thrombus distribution and endoleak.
Dissection of the Thoracic Aorta
Acute aortic dissection is a life-threatening medical emergency requiring prompt diagnosis and treatment. The 14-day period after onset has been designated as an acute phase because the rates of morbidity and mortality are highest during this period. The two most frequently used classifications (DeBakey and Stanford) are based on the anatomic location and extension of intimal flap ( Fig. 43.5 ). DeBakey’s nomenclature is based on the anatomic site of the intimal tear and the extent of the resulting dissection. In a type I dissection, the intimal tear originates in the ascending aorta, and the dissecting hematoma extends past the origin of the left subclavian artery, whereas type II dissections are confined to the ascending aorta. Type III dissections begin after the origin of the left subclavian artery and extend distally. The Stanford classification is conceptually founded on prognostic grounds, where type A involves the ascending aorta ( Fig. 43.6 ), regardless of the site of the entry tear, and type B dissections spare the ascending aorta and often imply a better prognosis. In general, acute dissections of the ascending aorta require emergency surgery, whereas descending aortic dissections may be managed with medical therapy and stent-grafting; in any case rapid diagnosis of the dissecting process and a delineation of its anatomic details are critical for successful management. The primary imaging goal is not only to establish the diagnosis of dissection, but also detection of entry and reentry sites, presence and degree of aortic insufficiency, and the flow pattern in the true and false lumen as well as in critical aortic branches. The latter is crucial for patient selection for transcatheter endovascular repair of type B acute and chronic aortic dissection as an alternative to open surgery. For diagnostic purposes the true lumen can be differentiated from the false lumen by the anatomic and functional (flow-related) features. In addition, the visualization of remnants of the dissected media as cobwebs adjacent to the outer wall of the lumen may help to identify the false lumen. The leakage of blood from the descending aorta into the periaortic space, which can appear with high signal intensity and can result in a left-sided pleural effusion, is usually better visualized on axial images. A high signal intensity of a pericardial effusion indicates a bloody component and is considered to be a sign of impending rupture of the ascending aorta into the pericardial space. A detailed anatomic map of aortic dissection must indicate the type and extension of dissection and distinguish the origin and perfusion of branch vessels from the true or false channels. In stable patients, adjunctive gradient echo sequences or phase contrast images can be instrumental in identifying aortic insufficiency and entry or reentry sites, as well as in differentiating slow flow from thrombus in the false lumen. The third step in the diagnosis of aortic dissection and definition of its anatomic detail relies on the use of Gd-enhanced 3D MRA. Because 3D MRA is rapidly acquired without any need of ECG triggering and Gd has minimal toxicity in patients with good renal function, this technique may even be used with severely ill patients. With spin echo sequences, artifacts caused by imperfect ECG gating, respiratory motion, or slow blood pool can result in intraluminal signal, simulating or obscuring an intimal flap. In Gd-enhanced 3D MRA, the intimal flap is easily detected, and the relationship with aortic vessels is clearly depicted. Entry and reentry sites appear as a segmental interruption of the linear intimal flap on axial or sagittal images. The analysis of MRA images should not be limited to viewing MIP images or surface-shaded display; it should also include a complete evaluation of reformatted images in all three planes to confirm or improve spin echo information and exclude artifacts. Combining the spin echo with MRA images completes the diagnosis and anatomic definition. At present, CMR is one of the most accurate tools in the detection of aortic dissection. A high degree of spatial resolution and contrast, and the capability for multiplanar acquisition provide excellent sensitivity and specificity rating at approximately 100% in the published series.
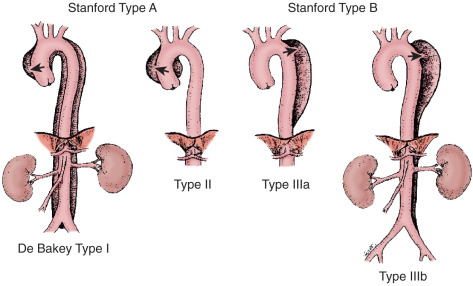
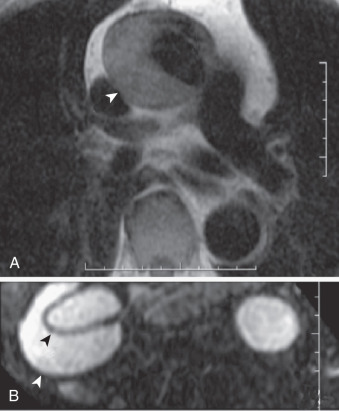
In selecting the diagnostic modality of choice, begin by considering what diagnostic information needs to be obtained. First, any chosen study must confirm or refute the diagnosis of dissection. Second, the study must determine whether the dissection involves the ascending aorta (type A) or is confined to the descending aorta (type B). Third, a number of anatomic features of the dissection should be identified, including its extent, the sites of entry and reentry, the presence of thrombus in the false lumen, the extent of branch vessel involvement, the presence and severity of aortic insufficiency, the presence of a pericardial effusion, and the presence of coronary artery involvement. It is also important to consider the accuracy of the diagnostic information obtained because a false-negative diagnosis may result in avoidable death, whereas a false-positive diagnosis might lead to unnecessary surgery.
According to the present information, CMR and TEE are the most sensitive modalities, with both performing better than aortography. The sensitivities of aortography, CT, and CMR are all quite high, whereas the specificity of TEE may be comparable only when a strict definition of a positive study is applied. Finally, availability, speed, safety, and cost should be taken into consideration in comparing various modalities. Aortography is rarely immediately available, requires transport of the patient, is a lengthy study, has the associated risks of both an invasive study and intravenous contrast, and is the most expensive. Yet it may be necessary in selected patients who are being considered for combined surgical treatment, especially those with a high likelihood of coronary artery disease or evidence of involvement of major arterial trunks arising from the aorta. CT scanning has the advantage that it is more easily obtained in less time and is noninvasive, but it is overall less accurate than the other techniques. CMR is usually less available in most hospitals, requires transportation of the patient, and is considered undesirable for unstable patients or those requiring very close monitoring. Meanwhile, TEE is readily obtained, quick to complete at the bedside and thus ideal for unstable patients, and is the least costly of the four imaging techniques. These options render angiography obsolete in the diagnostic workup of aortic dissection. Thus detecting dissection of the thoracic aorta should be a noninvasive strategy using CT or CMR in hemodynamically stable patients and TEE in patients who are too unstable for transportation. Comprehensive and detailed evaluation can thus be reduced to a single noninvasive imaging modality in the evaluation of suspected aortic dissection.
Although CMR may be less practical than CT and TEE to evaluate patients presenting with suspected aortic dissection, it is well suited for patients with stable chronic dissections. The extraordinary accuracy of CMR and the optional functional images may render CMR the gold standard for defining aortic anatomy and risk of rupture in dissection patients; for surveillance of dissection, CMR has already been widely accepted regardless of medical treatment, surgical repair, or endovascular management.
CMR can be used to custom design an individual stent graft for lesions such as aneurysms, aortic dissections, and localized aortic ulcers. With customized aortic endoprosthesis, such entities are becoming more frequently an ideal target for interventional treatment rather than surgical approaches that have high mortality and morbidity ( Fig. 43.7 ).
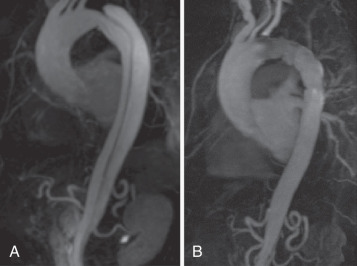
Aortic Intramural Hematoma
An important differential diagnosis of aortic dissection is intramural hematoma (IMH), which usually presents with the same clinical picture and risk profile as overt aortic dissection. Tomographic modalities such as CMR may be used to identify IMH with no luminal component, which is considered an imminent precursor of aortic dissection. With high-resolution tomographic imaging, the imaging diagnosis of IMH is now feasible, suggesting IMH as a precursor of dissection, with a 30% progression to overt dissection. Because of these findings in vivo before death, IMH appears more likely to be a variant of dissection than a separate entity. Typical epiphenomena of dissection such as aortic insufficiency, pericardial or pleural effusion may also occur in IMH. Spontaneous rupture of aortic vasa vasorum, especially of nutrient vessels to the media layer, has been suggested to initiate the process of aortic disintegration without an intimal tear. With a pathogenesis that explains the high rate of progression to overt aortic dissection and a prognosis and survival that are similar to those in aortic dissection, urgent diagnosis of IMH is very important.
The diagnosis of IMH relies on the visualization of intramural blood and/or evidence of localized increased wall thickness. CMR techniques, however, not only visualize the blood in the wall, but also allow an assessment of the age of the hematoma based on signal changes caused by the formation of methemoglobin ( Fig. 43.8 ). Acute IMH (early stage) is well imaged on T2-weighted spin echo images because of high initial signal intensity of blood, whereas blood of 1 to 5 days of age has lower signal intensity on T2 images. High signal intensity within the aortic wall on T1 spin echo images suggests subacute IMH, whereas acute IMH may be determined on T1 images from the isodense appearance of blood and aortic wall. Although TEE has an excellent sensitivity to detect aortic dissection, the definite distinction between IMH and normal findings may require a second tomographic modality such as CT or CMR, because a false-negative result (or false exclusion of IMH) is more likely to be avoided with independent morphologic information. In conclusion, as a precursor of dissection, IMH requires diagnostic attention by use of high-resolution tomographic imaging; owing to its physical properties, CMR may play a prominent role not only to diagnose IMH, but also to assess its age and to differentiate IMH from mural thrombosis; angiography certainly is not diagnostic in the setting of IMH.
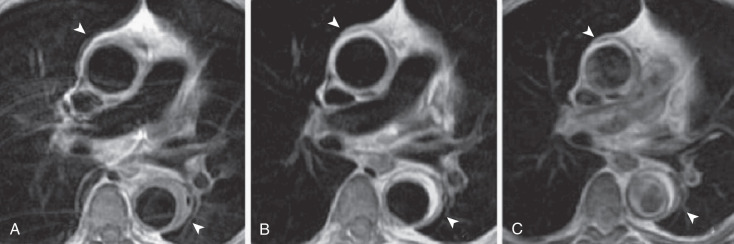
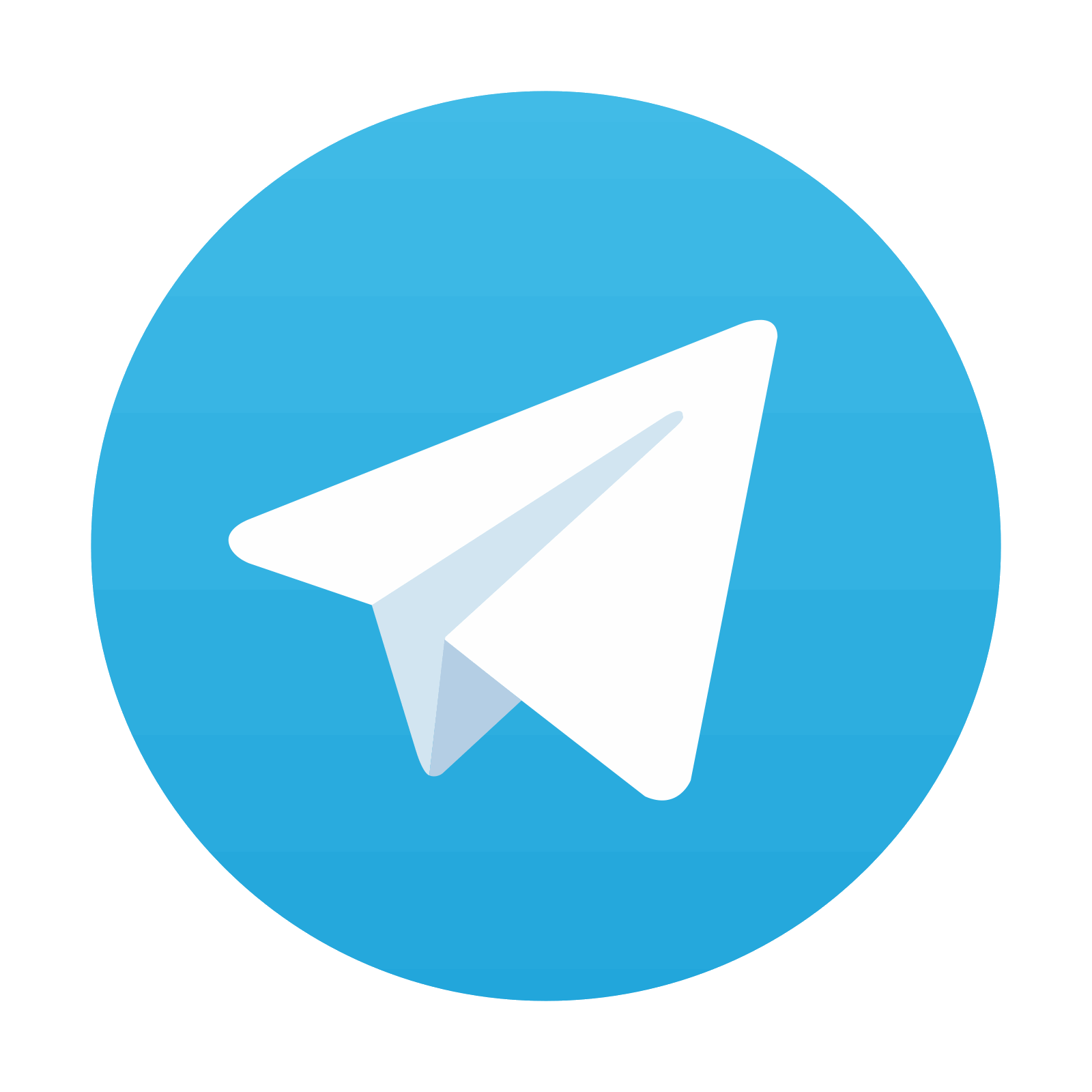
Stay updated, free articles. Join our Telegram channel
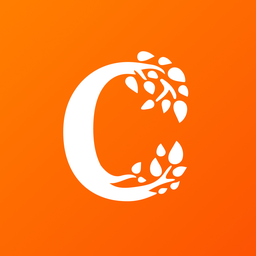
Full access? Get Clinical Tree
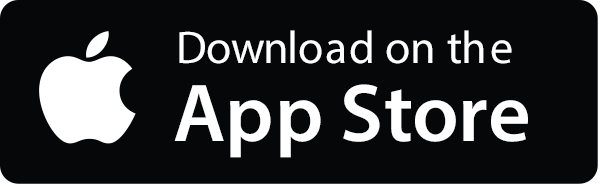
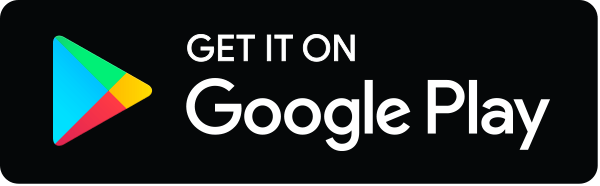
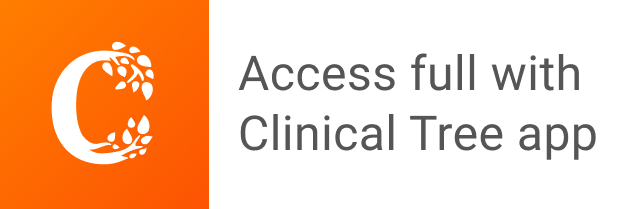