Fig. 8.1
Dr Raymond Kjellberg (and assistant) manipulating the stereotactic frame for a proton radiosurgery patient (left). An example dose calculation (not for this patient) shows the dose hand calculation for a pituitary lesion using 14 proton beams that penetrate beyond the target volume, but their composite dose creates a focal region with sharp penumbra
A second program for eye treatments commenced under the direction of Dr. Evangelos Gragoudas from the Massachusetts Eye and Ear Infirmary in Boston. Again, the static and visually apparent anatomy of the eye and neoplasm afforded effective use of localized proton radiation.
Both programs were effective without the use of volumetric imaging or dose calculations; both were sufficiently served by manual calculation processes.
A third program in large-field “conventional” radiotherapy commenced in 1974 under the aegis of Dr. Herman Suit from the department of radiation oncology at Massachusetts General Hospital. The introduction of this program did require the use of volumetric data sets. Dr. Michael Goitein was one of the first to combine volumetric image data provided by the now available CT scanners and computational algorithms in a treatment planning system, dubbed Rx, for proton radiotherapy. Rx presented the physician and physicist with the necessary information and confidence to treat internal neoplasms reconstructed from the volumetric data.
All three programs continued at the HCL until 2001 and were transferred, uninterrupted, to the Northeast Proton Therapy Center (now the F.H. Burr Proton Therapy Center) on the campus of the Massachusetts General Hospital, the second (the first was Loma Linda CA) proton facility within a hospital.
8.2 Implication for Modern Radiotherapy
The precision of proton beams requires concomitant precision in treatment planning capabilities, in treatment delivery, and in patient positioning. Thus, proton radiotherapy in the 1980s already required and implemented these now assumed obvious requirements for precision radiotherapy. The limitation in proton energy up to 160 MeV at the HCL necessitated a focus on precisely those neoplasms that proved particularly suited for early application of proton radiotherapy: those in the head and neck, in the cranium, and soft tissue sarcomas. It is indisputable that proton radiotherapy, as demonstrated in those sites and especially for chordomas, proved the axiom of radiotherapy: increasing dose while sparing normal tissue increases cure. It should be noted that the HCL did not, per se, demonstrate the superiority of protons. It primarily demonstrated that when precision in dose delivery is achieved, outcome can be improved. It secondarily demonstrated that protons well achieve such precision.
It was the well-understood physics of proton interactions in matter, i.e., scatter and energy loss, that allowed the precise manipulation of scattered and modulated (in energy and intensity) protons by mechanical means to create spread-out Bragg peak, SOBP, fields of variable range, and modulation. The use of apertures and range compensators, as modeled in the Rx treatment planning system, provided highly conformal 3D SOBP dose fields where the aperture served to provide lateral conformance, as in photon fields, and the range compensator served to provide distal target conformance to “stop” the proton field beyond the distal surface of the target.
Proton radiotherapy at HCL in the early 1980s was in sharp contrast to the parallel practice of radiotherapy. The first relied on now assumed obvious practices in precision therapy (Fig. 8.2), while the latter relied on X-ray simulation and simple 2D calculation methods. It would take two decades until photon radiotherapy would “catch up” and achieve the clinical performance of 1980 proton radiotherapy. Our question now is whether photon radiotherapy can maintain parity to proton radiotherapy to further the modern aims of radiotherapy.
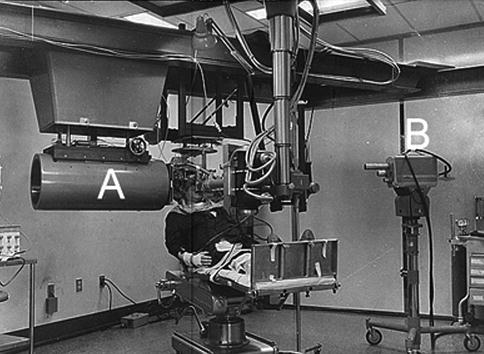
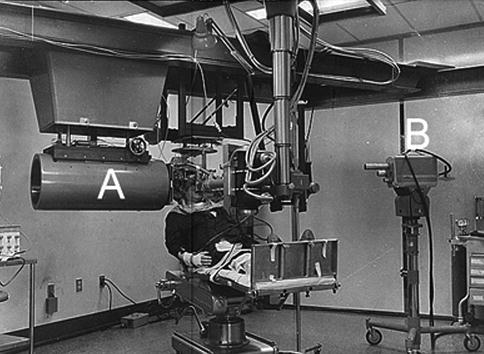
Fig. 8.2
Patient setup and verification in the stereotactic treatment room at the Harvard Cyclotron Laboratory. A water-filled variable range shifter (A) permitted variable penetration into the cranium, while X-ray (B) permitted setup verification. The treatment technology thus implemented now assumed standards of precision
8.3 Aims of Modern Radiotherapy and Proton Radiotherapy
Modern radiotherapy aims to optimize the response to radiation by minimizing dose to non-involved tissue to decrease normal tissue complications and to increase in target dose. These aims require improved differential imaging and identification of inter-target disease, improved localization (even in real time) with on-treatment imaging and target dose modulation, improved patient-specific response, and, now most importantly, improved quality of life for the patient.
These requirements require the application of in vivo and biological imaging capabilities, more advanced treatment planning and delivery systems, and, significantly, better understanding and utilization of differential biological response in the target and healthy tissues. Only some of these are furthered by external beam radiotherapy in the treatment management of the patient. All, however, need to synchronize over the course of treatment. It is this synchronization that is not promoted by the current treatment planning architectures. Instead, treatment planning for modern radiotherapy must be deployed in an architecture that permits its functions to be distributed and accessed in their appropriate operational location. Current treatment planning systems are inconsistent with this requirement.
Radiotherapy operates in a safe zone of dose fractionation imposed by dose toxicity of the, at least originally, large volumes of irradiated healthy tissues. Modern conformal dose treatments reduce the volume of irradiated healthy tissues and offer the opportunity to increase fraction doses and modulate dose within a target. If such opportunity benefits the patient, proton and ion radiotherapy outperform photon radiotherapy.
It is recognized that physical, chemical, and genetic regional differences can exist within a single target. It is assumed that in such regions, differential dose delivery in terms of local ionization differentials (i.e., different lineal energy transfer, LET, distributions) can improve response through enhanced local biological response. Ion radiotherapy has a twofold advantage compared to photon radiotherapy. The application of different doses to different regions within a target will benefit from that modality that can achieve the sharpest regional dose gradients. The use of modulated LET distributions can only be achieved with ion radiotherapy. Thus, proton and ion radiotherapy outperform photon radiotherapy where spatial and biological differentials are expected to improve outcome.
Imaging requirements prior and during treatment are core to the aims of modern radiotherapy. The original workflow model assumed that the treatment planning process preceded and remained static over a set of treatment delivery sessions. Treatment planning occurred as a stand-alone activity whose result was the treatment plan and the patient setup reference geometry to be applied and referenced daily. The daily effort to minimize inaccuracies and achieve presumed compliance was to reposition the patient to this static representation. Thus, the mitigation of all inaccuracies, and assumed worst case, had to be incorporated in the treatment plan to achieve acceptable dose coverage (and avoidance) over the time course of treatment. This process led to the definition of the planning target volume (PTV) as a geometric expansion of the target. This PTV expansion sufficed for photon treatments as the photon dose distribution in patient is invariant with respect to the patient’s anatomy and geometric setup which equates dosimetric accuracy. An PTV expansion, or a pure geometric registration of the patient, does not suffice in proton treatments as the dose distributions is sensitive to geometry, and thus, geometry and dosimetry are strongly coupled, and one cannot serve as a surrogate for the other. Whereas geometric setup certainly serves as the best first corrective action, it does not in and of itself guarantee dosimetric accuracy even in XRT.
The optimal process re-images and re-plans the patient before treatment delivery and monitors during treatment for compliance to the adapted plan (and in the furthest extreme adapts the radiation field during treatment). Particle beams in these processes offer novel capabilities compared to photon beams. The particle beam, as a unit or as individual particles, can be readily detected in task-specific detection systems. These include geometric ionization chambers for position, solid state imaging planes for indirect spectroscopy of particle interaction products, and Faraday chambers for energy. These unique (compared to a photon beam) methodologies offer unique opportunities to obtain necessary inpatient information during treatment and can enable real-time feedback in the delivery process.
It is important to observe that the control parameters in ion radiotherapy – per spot charge position and energy – are more consistent than those – MLC leaf positions – in photon radiotherapy. For the latter, these leaf positions are an awkward (if necessary) transformation from the physical quantity – fluence – in patient. For ion radiotherapy, the spot parameters represent fluence in patient directly, are the parameters that are used to control the delivery, and are the parameters that are observed directly. There is no transformation from the intended to observed quantities, and treatment delivery observation can be directly translated to the dose in patient and used in a feedback control to maintain the correct dose in the patient under variable circumstances. Thus, particle radiotherapy can achieve better delivery performance when considering the modern aims of radiotherapy.
Effective use of particle radiotherapy is hampered by its label “expensive.” Any therapy has to be of course cost-effective. This is, unfortunately, a subjective debate within a society and between societies. Nevertheless, if considered as a debate in terms of cost-benefit in development, deployment, and clinical effectiveness, the outcome should favor particle radiotherapy as many of the aims are better (and thus likely cheaper) achieved with particle radiotherapy. Looking back, of course, one should question that because proton radiotherapy in the 1980s was superior to photon radiotherapy and because the cost to achieve parity between the two modalities has been significant over three decades, perhaps we should have adapted protons more broadly earlier. We should pose the same question now where significant expenditures will be made to achieve the latest aims of radiotherapy and where ion radiotherapy may significantly improve on the achievement and efficacy of those aims.
8.4 Requirements for Treatment Planning
It is necessary to challenge the current model for treatment planning given the above stated aims and the necessary integration of up-to-date technologies in the treatment management processes. There are three facets in the current model. The first continues to emphasize the pretreatment static (over time) treatment plan model without explicit consideration of the dynamics of the possible daily changes in treatment or other changes incurred by clinical realities. The second perpetuates the deployment of treatment planning systems with a 1980s model of a “workstation” with a functionally overburdened software application that aims to achieve too many workflow steps within its confines. The third emphasizes algorithmic components but not computational architectures that promote data management, communication, and workflow processes.
The deployment of a single, heavy, and shrink-wrapped application makes it difficult and contrived to move the various functions that accommodate the adaptive radiotherapy workflow to their optimal locations. In contrast, modern computing paradigms emphasize service-oriented architectures that promote logical decomposition of computational and data management domains and promote distributed access to these services. These architectures are based on distributed and disjointed processes connected by communication protocols.
Of particular interest are radiotherapy data management requirements characterized by large data sets and numerous temporal, logical, and computational associations between data. For example, a dose computation result should adapt when a treatment field parameter changes. The consequences of time have to be incorporated to model both motion effects and to model changes over the course of treatment.
Data management for radiotherapy has been often managed at the operating system file system level where individual patients are mapped to directories and patient data to sub-directories and files. Associations between data (i.e., a beam dependency of a dose calculation) were typically not represented or implicit, and the state (i.e., the dose up to date vis-à-vis the beam state) was assumed. Relational databases have been commercially popular and readily available but are ill suited to radiotherapy data representations. Relational databases impose a rigid schema structure on the data and are coded to perform relational queries on large datasets of the type which are not useful for radiotherapy data (i.e., one is generally not interested in finding all patients with a gantry angle between 0° and 10°). Thus, neither the data casting nor the framework and its significant overhead are useful.
Instead, radiotherapy data is best managed by hierarchical structures linked by key-value association pairs to manage links between data instances. These key-value pairs and the desired query operations are better managed by much simpler systems such as NoSQL databases. Data is contained in XML documents, e.g., that allow for dynamic changes in the data definitions, dynamic management of associations, and ready replication and versioning. Most importantly, such databases readily scale to accommodate the problem and data size and promote distributed architectures.
Modern deployment models, such as cloud computing, are still absent in radiation oncology. It is clear, however, that the large computational requirements readily benefit from such models. A cloud deployment for computational and data management services would be to great benefit for both smaller and larger clinics. It provides affordable access to necessary computing resources on demand and distributed access in a hospital network. It frees clinical centers from the burden to invest in ever increasing computing hardware.
The limitations of the current, functionally monolithic, systems for treatment planning (TPS) and treatment management (TMS) have led to the introduction of ad hoc and institution-specific procedures to implement and manage workflow. After all, the clinical reality may require a patient to receive a new treatment plan which currently is essentially a repeat of the workflow for that patient’s treatment plan. Explicit tracking of such a repeat workflow, however, is not rendered in the TPS.
Of great significance is the work of the DICOM radiotherapy Working Group 7 which, in DICOM supplement 147, makes explicit the need for data objects that model the changing state of the patient as a consequence of treatment events and adaptable workflow tasks. In addition, IHE-RO, the Integrated Health Enterprise comprised of committees concerned with vendor and equipment interoperability and workflow within the radiation oncology domain, has defined specific workflow profiles that specify the details of various composite workflow processes necessary to accomplish tasks. An example workflow profile is “Integrated Positioning and Delivery Workflow,” which concerns itself with the positioning of a patient prior to treatment delivery, position monitoring (if any) during treatment delivery, and radiation delivery all managed by a single device.
In summary, modern requirements for treatment planning emphasize distributed computing as afforded by service-oriented architecture, explicit modeling of the workflow to dynamically connect the services needed as the treatment session unfolds.
8.5 Case Study
8.5.1 Volumetric Studies
Volumetric treatment planning requires the use of volumetric image studies for both photon (XRT) and proton radiotherapy (pRT). For pRT, the use of CT is axiomatic as CT is the only practical modality for which acceptable conversion from image voxel data, Hounsfield unit for CT, to voxel stopping power has been validated.
Volumetric image studies are necessary to define the physical computational space as represented by volumetric voxel elements and which allows the accurate computational transport of radiation through the patient’s anatomy.
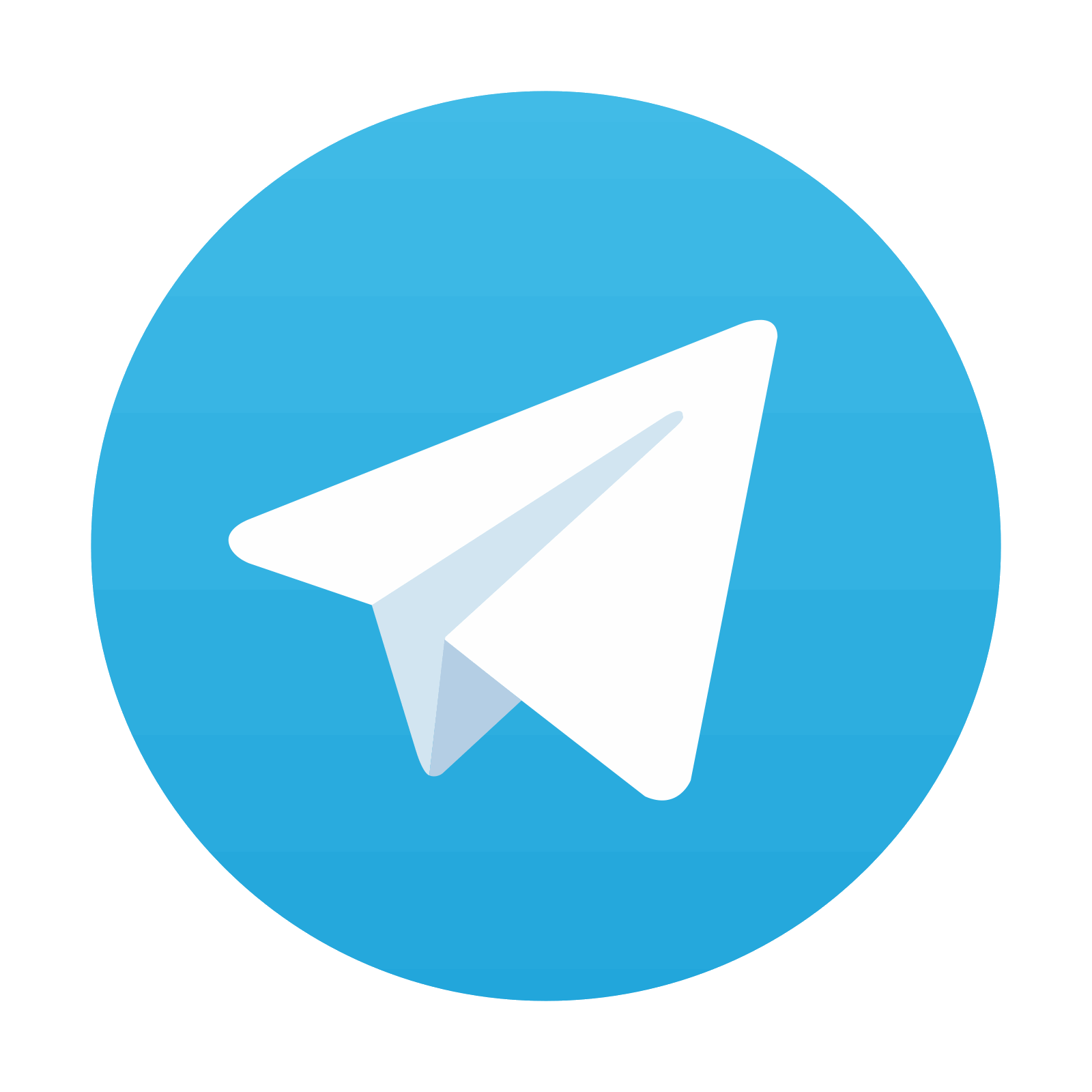
Stay updated, free articles. Join our Telegram channel
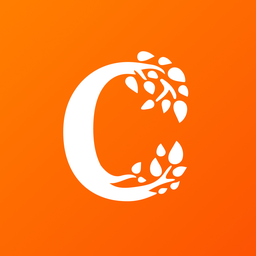
Full access? Get Clinical Tree
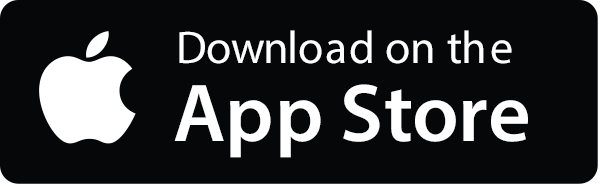
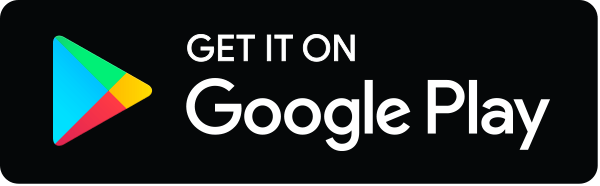