Fig. 2.1
Images obtained by mechanical 3-D acquisition. The top panel represents 3-D imaging of a plaque phantom with corresponding x-, y-, and z-plane sample acquisitions at the plaque level. The bottom panel illustrates a 3-D reconstruction with half-volume cutaway of a carotid artery bifurcation from a patient with lumen-encroaching plaque (From Graebe et al. [9], with permission). In the top figure, panel 4 represents the 3-D reconstruction with x-, y-, and z-planes represented in panels 1-3 to the right. In the bottom figure, arrows depict regions of plaque in different regions of the carotid including the common carotid (A), the bifurcation (B), and the internal (D) and external (C) carotid
2.1.3 Imaging Plaque Composition
There has been extensive study of what constitutes disadvantageous plaque biology with regard to likelihood for rapid progression or susceptibility to atherothrombotic complications such as stroke and acute coronary syndromes. Some of the high-risk morphologic or cellular characteristics of a plaque include eccentricity, inflammatory cell burden and cell type, necrotic core, high lipid or foam cell content, thin fibrous cap which is defined differently for carotid and coronary vessels, microcalcifications, evidence of recent intraplaque hemorrhage, cellular apoptosis, proliferation of the vasa vasorum or plaque neovessels, and plaque ulceration or erosion [10–12].
The most basic attempts to identify high-risk plaque phenotype have been based on detecting either echolucency of plaques which have been correlated with increased lipid and necrotic core content and plaque calcification which has been associated with enhanced inflammatory activity. In cross-sectional studies, these ultrasound features have been shown to be more prevalent in unstable than stable plaques, and more recently they have been shown to provide a modest estimate of risk in prospectively followed patients [4, 10, 13]. The presence of echolucent plaques in one location (e.g., carotid artery) has also been shown to be an indicator of greater disease burden in other vascular beds [14]. With regard to calcification, the presence of a high degree of coronary calcification for age has been demonstrated to be one of the most useful markers for reclassifying risk for patients after taking into account non-imaging risk factors [15]. Although with these findings and the demonstration on histology of the relation between calcium with inflammation, it is generally accepted that lack of calcium does not necessarily denote low risk, since lipid-rich lesions with thin fibrous cap may not have much detectable calcium particularly in young individuals.
The ability to produce robust images at high transmit frequencies and the increased computational power in imaging systems have resulted in techniques that have been able to discriminate tissue types beyond simply “echolucent” and “calcified” by advance radiofrequency spectral analysis (frequency spectrum, amplitude, extinction) of backscattered signals (Fig. 2.2) [11]. Such radiofrequency analysis can characterize tissues as fibrous, fibrofatty, necrotic, and calcified with high spatial resolution and can provide a modest measure to identify plaques that may have a high likelihood for progression or for atherothrombotic complications [11, 16, 17]. The ultrasound signatures that have been used to discriminate tissue type have been based on both modeling and radiofrequency signatures of tissue type from ex vivo vascular specimens. Some of the high-risk features of radiofrequency tissue characterization include fibrous cap thickness, volume or circumferential extent of necrotic tissue, and heterogeneity in tissue types. Although there are no prospective studies to suggest that the findings of these techniques can be used to select any therapy, they have been shown to provide a measure of risk for acute adverse atherosclerotic events particularly when paired with information on plaque size or disease burden [17].
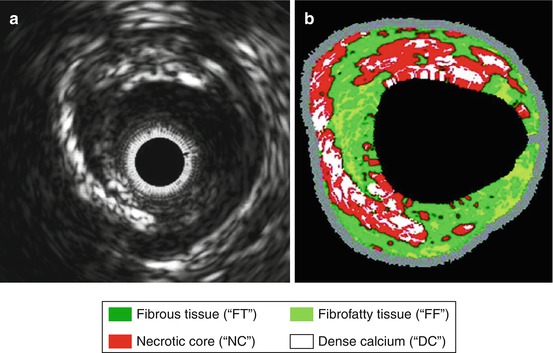
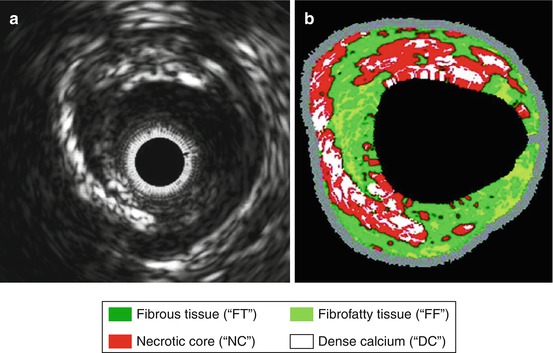
Fig. 2.2
B-mode ultrasound imaging of a coronary artery with eccentric plaque. (a) Gray-scale 2-D intravascular ultrasound image and (b) corresponding color-coded virtual histology image produced from radiofrequency spectral analysis demonstrating different plaque components according to the key at bottom (From Konig and Klaus [11], with permission)
2.1.4 Mechanical Properties
Mechanical properties are what determine the 3-D deformation of a vessel under various degrees and directions under stress. In turn, the mechanical properties are determined by the physical dimensions and composition of the vessel wall. Methods for globally assessing arterial mechanical properties in a rapid fashion have been developed, some involve ultrasound measurements such as pulse wave velocity (time delay in the onset of a systolic pulse from a proximal to more distal site in large conduit vessels) and elastic modulus which reflects stress-strain relationships by any number of mathematical approaches to correlate pulse pressure to the change vascular dimension [18, 19]. Methods for spatial quantification of arterial mechanical properties have been developed but are still investigational. One approach has been to use either Doppler data or speckle-tracking echo to measure vascular strain (the degree radial or circumferential deformation) or strain rate [20–22]. This can be done in a transmural approach or just for the immediate luminal region which is sometimes referred to as palpography (Fig. 2.3) [20, 23, 24]. These approaches have been used to detect reduced vascular compliance due to longstanding hypertension [18]. They have also been used in a global or regional fashion to detect increases in vascular distension that occur in high-risk plaque due to inflammatory response, necrosis, and abnormal matrix homeostasis or in patients with high risk for unstable aortic complications from medial aortopathy (Marfan’s, bicuspid aortic valve) [20, 21, 24, 25]. In particular regions with high heterogeneity of strain have been correlated with high risk for vascular rupture [24, 26, 27]. These findings suggest that shear rates within the vessel wall may be as important as shear rate at the luminal surface. Some of the limitations of the technology include that it cannot be performed for coronary vessels noninvasively, lack of studies evaluating 3-D or longitudinal strain patterns, and the inability to accurately measure focal stress (pressure) which can be influenced flow streams that become non-laminar from eccentric stenosis or at arterial branch points.
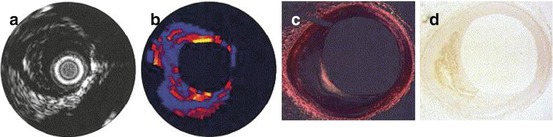
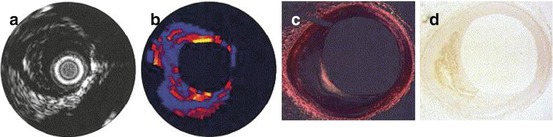
Fig. 2.3
Correlation of B-mode and elastography ultrasound with histology in a coronary artery with eccentric plaque. Ultrasound images include (a) intravascular ultrasound 2-D imaging and (b) corresponding elastogram map with yellow areas demonstrating a region of high strain and blue areas demonstrating low strain. (c, d) Histology revealing a plaque with large volume, eccentric remodeling, and thin-cap atheroma (From Schaar et al. [24], with permission)
2.1.5 Vasa Vasorum and Plaque Neovessel Imaging
Expansion of the vasa vasorum (VV) and development of plaque neovessels that penetrate into the tunica media and intima have been causatively linked with risk for unstable atherosclerotic progression and plaque rupture [28]. These neovessels may promote instability in a number of ways. They can be a portal of entry for immune cells, lipoproteins, platelets, and erythrocytes that contribute to adverse plaque composition and inflammation. Conversely, VV may also be a reflection of high-risk plaque phenotype, since the growth factors, cytokines, and proteases that mediate neovascularization are in part triggered by the inflammatory response, intraplaque hemorrhage, and/or by oxidative stress [29].
While the density of VV and plaque microvessels on histology can be very high, often very few of these vessels are actively perfused at any one time. This functional inactivity and the very slow flow velocity in vasa vasoral vessels make them very poorly amenable to detection by standard Doppler-based techniques. More recently, ultrasound contrast agents which are composed of acoustically active encapsulated microbubbles have been applied to characterize the degree of plaque neovascularization. These contrast agents are approved for use in many countries for left ventricular cavity opacification on echocardiography and for liver imaging to better discriminate intrahepatic masses [30]. The degree of neovascularization in severe carotid artery plaques has been shown to be higher in those with unstable versus stable plaques based on clinical presentation and has been shown to correlate well with the number of plaque neovessels on histology from carotid endarterectomy specimens [31, 32]. The degree of plaque neovascularization on noninvasive contrast-enhanced ultrasound has also been shown to be greater in plaques that are echolucent [32, 33]. This finding is not unexpected, since the density of vessels making up the vasa vasorum by histology has been shown to increase with macrophage density and to be higher in plaques with thin-cap fibroatheromas rather than stable plaques [12].
A finding common to all studies that have compared contrast ultrasound to histology is that the degree of plaque neovascularization is much greater than one would predict from the contrast ultrasound enhancement. This finding likely reflects the low fraction of vessels that are actively perfused at any time and/or the very low blood flow through the vasa vasorum network. As a consequence, microbubble transit through plaque neovessels is in most circumstances an infrequent event compared to what is seen in the blood pool adjacent to the plaque surface. Because the presence and extent of plaque neovessels is probably more important than network flow, maximum intensity projection processing has been used which provides a more robust method for detecting these vessels even if flow and/or microbubble concentration is low (Fig. 2.4) [34, 35].
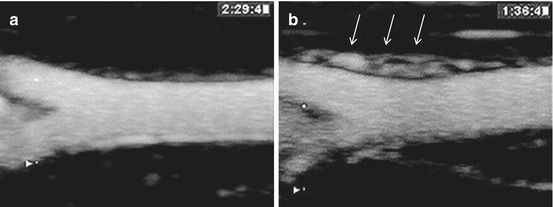
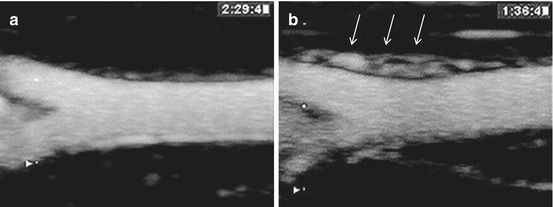
Fig. 2.4
Contrast-enhanced ultrasound imaging of rabbit femoral arteries with maximum intensity projection processing. (a) Normal femoral artery with minimal vaso vasorum. (b) Femoral artery in an atherosclerotic rabbit model of vasa vasoral proliferation (arrows) (From Lee et al. [34] with permission)
2.2 Ultrasound Molecular Imaging of Atherosclerosis
Molecular imaging of components of atherosclerotic plaque has become possible for all forms of noninvasive medical imaging. This advance has been based primarily on the creation of customized targeted imaging probes, although innovations in detector technology have also been important. Although a clinical application is yet to be established, molecular imaging of atherosclerosis could potentially be used to detect disease at an early and more “intervenable” stage, provide unique prognostic or pathophysiologic information that can be used to select or guide therapy, or to evaluate response to therapy. In the research realm, molecular imaging is already beginning to play an important role in the investigation of new pathophysiologic mechanisms that can be targeted for therapy or for the high throughput testing of new targeted therapies.
Key considerations when choosing a molecular imaging technique are whether it has adequate sensitivity to detect early events, whether it has adequate resolution to resolve spatial heterogeneity, and whether it is selective for the pathologic event of interest [36]. Molecular imaging of atherosclerosis with ultrasound has been used in the research realm primarily because it offers a balance between spatial resolution and sensitivity, multiple targets can be evaluated in a short imaging timespan, and the technology required to perform imaging is relatively inexpensive.
2.2.1 Basics of Ultrasound Molecular Imaging
2.2.1.1 Ultrasound Contrast Agents
Nontargeted contrast-enhanced ultrasound imaging relies on the ultrasound detection of acoustically active microbubbles or nanoparticles. Ultrasound contrast agents are currently used clinically in many countries for delineating the blood pool during echocardiography, enhancing Doppler signals, and characterizing vascular patterns and phagocytic properties in the liver [37, 38]. Ultrasound contrast agents are also used for off-label indications such as the evaluation of myocardial or tumor perfusion [39].
Ultrasound contrast agents are typically composed of gas-filled encapsulated microbubbles, liposomes, or nanoparticles. All agents that are approved for human use are composed of microbubbles that are filled with a high molecular weight gas (perfluorocarbons or sulfur hexafluoride) and have a shell composed of lipid or albumin which enhance stability. These agents are generally around 1–3 μm in diameter and do not coalesce after in vivo injection. For most vascular beds, these contrast agents are confined to the vascular compartment and they possess a rheologic profile similar to that of RBCs [40]. Accordingly, these agents transit through the microcirculation of most organs unimpeded. In general, most microbubbles are removed from the circulating blood pool by reticuloendothelial organs within 5–8 min [41, 42].
When exposed to ultrasound, microbubbles undergo volumetric oscillation with compression and expansion during the pressure peaks and nadirs of the ultrasound field [37, 43]. When stimulated at or near their ideal resonant frequency (which is determinated by size and shell properties), microbubbles will undergo nonlinear oscillations which produce harmonic signals (ultrasound emissions at multiples of the transmission frequency). These harmonic signals are used to enhance ultrasound imaging by increasing signal to noise ratio [44]. The tissue is less compressible and therefore produces fewer nonlinear signals and can be distinguished from the nonlinear signals generated from the microbubbles particularly when using new multipulse signal subtraction techniques that eliminate most linear signal. High acoustic pressure amplitudes result in exaggerated microbubble oscillation and subsequent microbubble destruction which is termed inertial cavitation. When this occurs, there is often formation of transient free microbubbles which also produce strong and broadband acoustic signals. Many factors affect the cavitation properties of microbubbles including shell properties, gas core compressibility, microbubble radius, temperature, and viscosity of the surrounding medium [37].
2.2.1.2 Targeted Microbubble Contrast Agents
Microbubbles can be targeted to disease-specific ligands to characterize disease phenotype. Targeting can be achieved by either chemical modification of the microbubble shell or direct attachment of ligands (e.g., antibodies, peptides, glycoproteins) to the microbubble surface which usually also involves a molecular spacer in order to reduce steric hindrance during cell-microbubble interaction (Fig. 2.5) [36, 37].
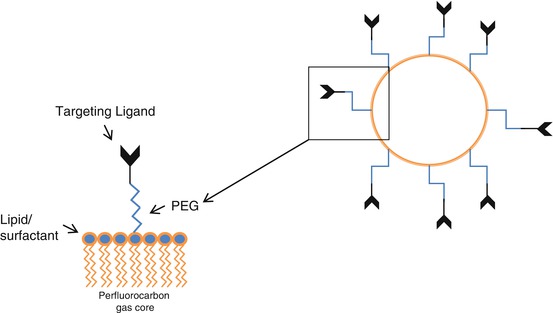
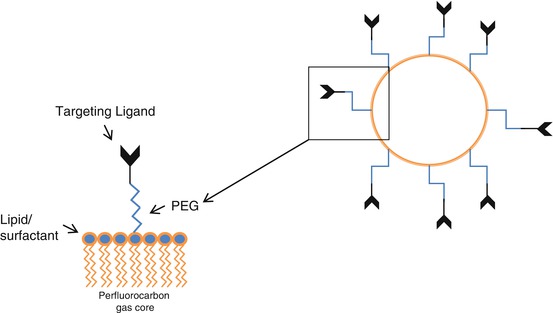
Fig. 2.5
Typical composition of targeted lipid-stabilized perfluorocarbon microbubble agent
Albumin- and lipid-shelled microbubbles are capable of attachment to activated leukocytes or even activated endothelial cells via integrin or complement-mediated interactions (Fig. 2.6) [45]. The addition of negatively charged lipids, in particular phosphatidylserine, to the microbubble shell is a simple chemical modification that can amplify complement-mediated interactions for lipid-shelled agents [37, 45–47]. These nonspecific cell-microbubble interactions result in some degree of background signal enhancement when imaging inflammatory processes with specific ligand-directed ultrasound contrast agents.
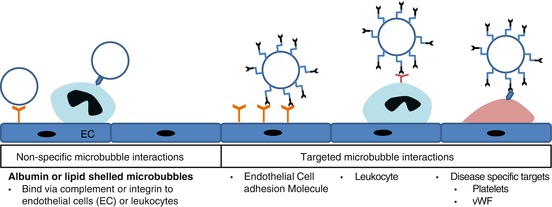
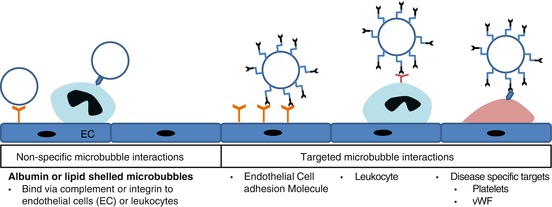
Fig. 2.6
Potential mechanisms for microbubble retention from endothelial attachment in inflamed tissues
Specific molecular targeting is achieved through surface conjugation of ligands to the surface of microbubbles or nanoscale contrast agents. In general, several thousand ligands per square micron are conjugated to the cell surface [47, 48]. Accordingly, contrast ultrasound imaging does not rely on one-to-one relation between targeted molecule and imaging tracer, but rather relies on attachment of a multivalent particle in a fashion that in many ways mimics the recruitment of circulating blood cells to the vessel surface.
Successful targeting of ultrasound contrast agents depends upon multiple considerations. For atherosclerosis imaging, these considerations include: (1) selection of a targeting ligand with high affinity and specificity for the target, (2) selection of a target molecular that is specific for the disease process of interest, (3) selection of a target molecule that is accessible by a pure intravascular tracer such as microbubbles, (4) sufficient stability of agent to generate a strong acoustic signal after attachment to target, (5) the ability to bind in vessels characterized by high shear forces, and (6) the ability to differentiate signals that come from attached versus freely circulating microbubbles [36, 49, 50]. The most important challenge when designing ultrasound molecular imaging agents is not in the preparation of the imaging probe, but rather the selection of appropriate high-performance targeting ligands that are able to bind with sufficient bond force despite the presence of endogenous competitive ligands. With regard to stability, freely circulating microbubbles are generally cleared within 5–10 min after intravenous injection. Studies have confirmed that retained microbubbles are not only able to retain their acoustic properties over this course of time but are not substantially damped through the process of attachment [51].
2.2.1.3 Targeted Contrast-Enhanced Ultrasound Molecular Imaging
Microbubble signal on ultrasound imaging is generated either by stable cavitation (stable radial oscillation which produces fundamental and harmonic frequencies) or by inertial cavitation whereby microbubbles undergo destruction from exaggerated nonlinear oscillation and transient formation of free gas nuclei which can serve as cavitation nuclei which produces not only harmonic but broadband frequencies. The type of cavitation depends on both the ultrasound frequency and acoustic amplitude. On clinical imaging systems, the ultrasound power is often displayed as the mechanical index (MI) which reflects the peak negative rarefactional pressure divided by the square root of the transmit frequency. Most microbubble agents are destroyed at the high MIs that are frequently used during diagnostic imaging. Techniques have been developed to avoid destruction and to maximize signal-to-noise ratio through elimination of tissue signals [43, 44]. Both low and high mechanical index (MI) imaging protocols have been used with different applications for contrast ultrasound imaging. In general, high MI imaging techniques can be used when signal needs to be amplified due to low contrast density.
Targeted molecular imaging using contrast-enhanced ultrasound utilizes a single bolus intravenous injection of an optimized microbubble dose according to the organ of interest, the expected adhesion density, and the magnitude of signal expected according to ultrasound frequency and power. In order to allow clearance of freely circulating bubbles, imaging is usually initiated 5–10 min after the initial bolus injection. If imaging is not delayed, then the majority of microbubble signal from most organs is attributable to the freely circulating microbubble pool. The delay in imaging allows targeted attachment and clearance of freely circulating tracer [52]. The time delay is also dictated by the tissue that is imaged. For example, a short period of time is sufficient for an organ with a low blood volume and high expected retention fraction for targeted tracer. On the other hand, targeted imaging of atherosclerosis in large blood vessels requires detection of retained agent that is directly adjacent to the blood pool so that time delay must be sufficiently long for removal of most of the freely circulating tracer.
When imaging retained tracer, signal at low or high MI can be used. If high MI is used, only the first one or two frames can be used to for analysis due to subsequent destruction of agent. Regardless of the power used to record targeted microbubble signal, the contribution from freely circulating microbubbles can be eliminated through an algorithm that has been frequently employed for in vivo imaging. The initial imaging frame signal obtained at high or low MI includes total bubble concentration of freely circulating and adhered microbubbles. All microbubbles within the imaging sector can then be destroyed by high MI imaging. After destruction the mechanical index is returned to its initial setting and the images captured post-destruction represent freely circulating bubbles. Digital subtraction of post-destruction averaged frames from the initial pre-destruction frame then creates the targeted molecular signal [52, 53]. Because in certain organs, the number of microbubbles retained depends on blood flow (which determines total number of microbubbles fluxing through tissue), methods have been developed that are able to estimate retention fraction of microbubbles [54]. This approach employs continuous low-MI imaging and deconvolution of the time-intensity curve into a gamma-variate function to reflect those microbubbles passing through after a bolus without retention and an integral of a gamma-variate to reflect the population that is retained. Retention fraction is not necessarily germane to plaque imaging where most of the target molecules are directly adjacent to a large blood pool and, hence, flow is not a major determining factor of retention.
The methods for imaging targeted contrast ultrasound tracer discussed above have largely relied on external imaging using contrast-specific ultrasound detection schemes and low-to-medium frequency (<10 MHz). However, IVUS has also been used to assess molecular and cellular components of atherosclerosis. In these studies, echogenic liposomes or lipid-shelled perfluorocarbon nano-emulsion agents have been used based on a smaller size than microbubbles and higher likelihood to produce acoustic signal rather than attenuation at the high frequencies usually used during IVUS [55, 56]. Signal-to-noise ratios for all acoustically active agents are generally not very high for these frequencies, and, when adhered microbubble concentration is high, the degree of attenuation relative to signal enhancement is also somewhat unfavorable. Hence, newer techniques using lower frequency probes or even photoacoustic imaging are being explored.
2.2.2 Molecular Targets of Atherosclerosis Accessible to Ultrasound Contrast Agents
Target selection for molecular imaging requires clear purpose of how the information is to be used. Nowhere is this more important than in atherosclerosis where the molecular environment can vary according to stage of development. For example, evaluation for a “vulnerable plaque” or “vulnerable patient” defined as heightened susceptibility to acute atherothrombotic complication is best accomplished by imaging the specific processes that lead to plaque rupture or thrombotic occlusion. On the other hand, molecular imaging of future risk of aggressive disease decades before disease becomes symptomatic would rely on detection of events that lead to initiation and progression of plaque. For contrast ultrasound molecular imaging, the array of targets must also be honed to those that are accessible to the micro- or nanoscale contrast agents that are normally confined to the vascular compartment.
Endothelial activation is an early event in the development of atherosclerosis and plays a role in the progression of disease. One aspect of endothelial activation is the expression of adhesion molecules that participate in the recruitment of leukocytes from the blood pool [42]. Leukocyte recruitment involves a multistep process of cell capture, rolling, activation, adhesion, and transmigration [57]. All of these processes involve the cytokine-mediated expression and/or activation of endothelial cell adhesion molecules and leukocyte counter receptors. Initial capture and rolling involves primarily selectins on endothelial cells (P- and E-selectin) interacting with leukocyte glycoprotein counter receptors (e.g., P-selectin glycoprotein ligand-1 or PSGL-1) or leukocyte selectins (e.g., E-selectin). There are data that rolling can also be supported by fractalkine (CX3CL-1) on endothelial cells and its leukocyte receptor CX3CR-1, as well as endothelial vascular cell adhesion molecule-1 (VCAM-1) interaction with leukocyte VLA-4 (α4β1 integrin) [58]. Leukocyte rolling can lead to further activation and/or expression of integrins. Integrin interactions with endothelial cell adhesion molecules such as intercellular adhesion molecule-1 (ICAM-1) and VCAM-1 are largely responsible for leukocyte adhesion followed by eventual migration into the subendothelial space. These steps are critical in the recruitment of inflammatory cells to regions of atherosclerotic plaque.
The importance of imaging adhesion molecule expression is underscored by the importance of monocytes/macrophages and T-cells that are recruited by these molecules which participate in the initiation, growth, and destabilization of plaque [59]. Inflammatory cells are present at both early and late stages of atherosclerosis, but they are also found in high concentrations in ruptured plaques demonstrating not only their role in plaque initiation but also in plaque disruption. It has also been suggested that imaging of selective monocyte subtypes may be useful in evaluating higher-risk disease [59].
Defining and identifying plaque ruptures that do not lead to overt acute coronary syndrome may represent another approach for examining unstable plaque phenotype. At the time of plaque rupture or vascular injury, thrombogenic materials such as collagen, VWF, and fibronectin are exposed which triggers a cascade of events including platelet activation, adherence, and aggregation [60]. Platelets adhere to the damaged vessel and further aggregation occurs in a large part from glycoprotein GPIIbIIIa integrin cross-linking via fibrinogen. While the role of platelets in thrombotic complications of atherosclerosis is well defined, their role in early atherosclerosis is less understood. Platelets have been identified in early stages of atherosclerosis and may be involved in the inflammatory process that initiates plaque formation [61–63]. Three mechanisms by which platelets interact with the endothelium in situations other than plaque rupture include: (1) direct platelet-endothelial interactions such as via GPIb and Mac-1, (2) platelet-vWF interactions, or (3) platelet-leukocyte interactions which are largely mediated by P-selectin. Attachment of ex vivo platelets to atherosclerotic lesions has been detected in both atherosclerotic rabbit and apolipoprotein E −/− (ApoE−/−) mouse models [63, 64]. In the latter, reduction of platelet-endothelial interactions was associated with reduced lesion formation.
2.2.3 Ultrasound Molecular Imaging of Atherosclerosis
Ultrasound molecular imaging of endothelial cell activation and atherosclerosis can be achieved via two different approaches. The first relies on nonspecific interaction between microbubble contrast agents and either endothelial cells or leukocytes in tissues where there is inflammatory activation or by direct ligand-targeting approaches.
2.2.3.1 Non-ligand Microbubble Targeting
Early studies using myocardial contrast echocardiography perfusion imaging in postischemic tissues detected the persistence of microbubble signal [65]. It was subsequently shown that albumin- and lipid-shelled microbubbles adhere to activated leukocytes on the endothelium and are retained in areas of inflammation in proportion to the degree of inflammation [41, 45, 65]. Inhibitory studies demonstrated that albumin and lipid microbubble-leukocyte interactions are mediated by leukocyte Mac-1 (ß2-integrin) and complement dependent, respectively [65]. After demonstrating the adherence of microbubbles to leukocytes, further studies were done to define microbubble-leukocyte interactions. Transmission electron and intravital microscopy studies demonstrated that microbubbles can undergo phagocytosis by activated leukocytes which results in persistent albeit damped signal generation on ultrasound imaging [41]. In order to amplify complement-mediated interactions by lipid microbubbles, phosphatidylserine has been added to the shell which results in significant signal enhancement on myocardial and renal ischemia reperfusion.
Subsequent studies used these nonspecific microbubble interactions to assess atherosclerosis. In a swine model with balloon injury-induced carotid artery endothelial dysfunction, albumin microbubbles have been found attached not only to leukocytes but to the injured endothelium [66]. These microbubble-endothelial interactions were confirmed to be complement mediated [67]. Subsequent studies in several different animal models have demonstrated the direct binding of nontargeted albumin-shelled microbubbles to damaged endothelium at early to multiple different stages of disease. Despite the simplicity of using nonspecific interactions between microbubbles and endothelial cells, signal generated from these interactions is probably not sufficiently robust for clinical use. However, this background level of adhesion serves to explain the findings in many other studies of ligand-directed molecular imaging where “control nontargeted” microbubble signal in atherosclerosis is not negligible.
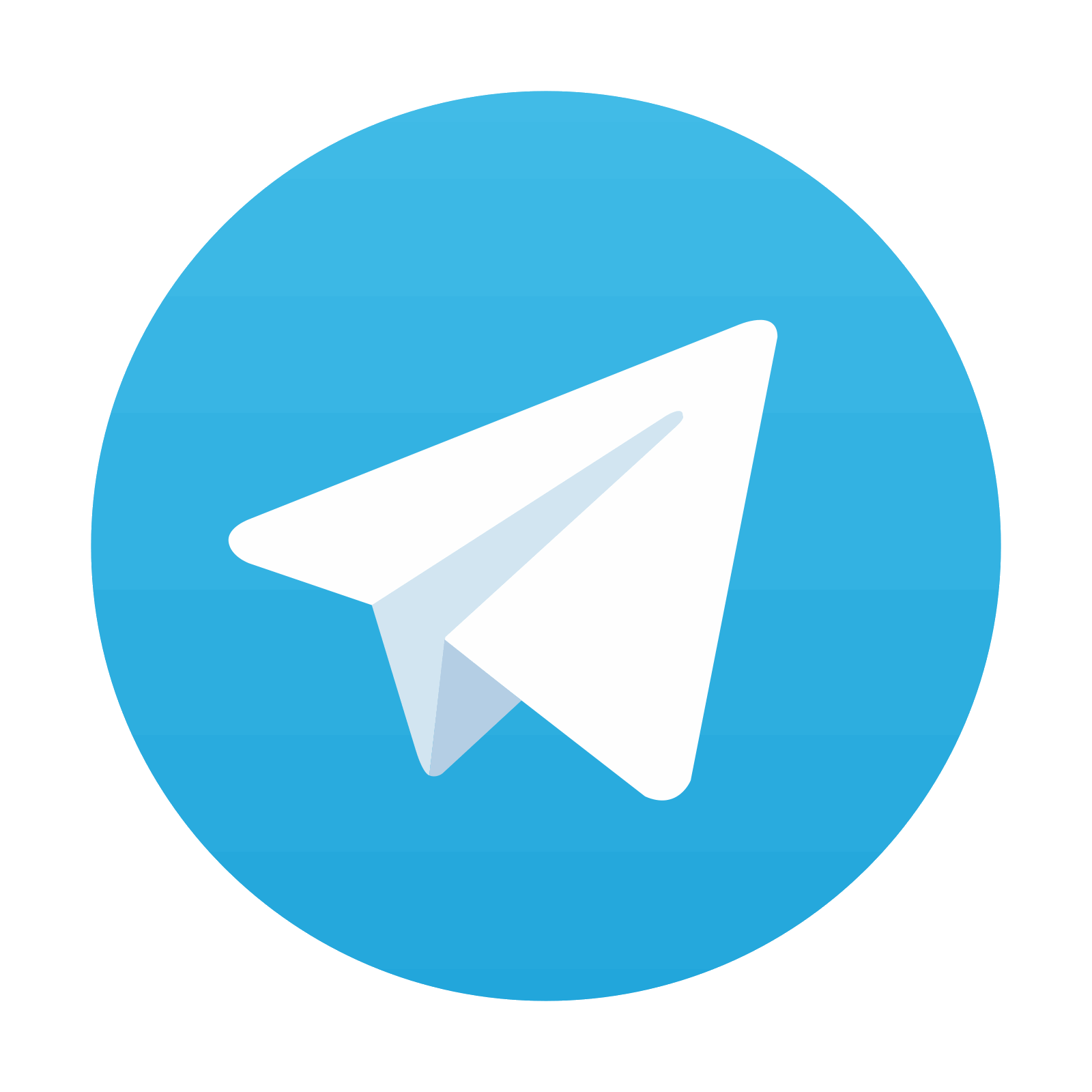
Stay updated, free articles. Join our Telegram channel
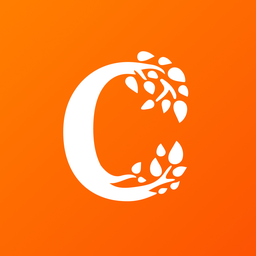
Full access? Get Clinical Tree
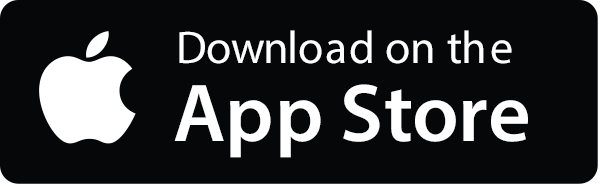
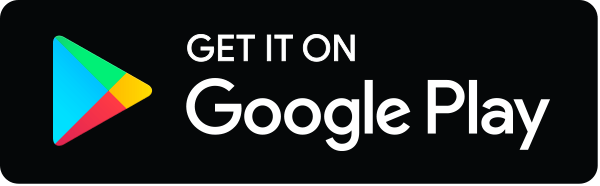