html xmlns=”http://www.w3.org/1999/xhtml”>
Chapter 16 Abusive head trauma: clinical, biomechanical, and imaging considerations
Introduction
Abusive head trauma (AHT) is a medical diagnosis that encompasses data from clinical and experimental studies. The neuroimaging evaluation is a critical piece of the diagnosis, but should not be considered separate from the biomechanical research, clinical history, laboratory testing, and any other investigative information that is felt to be clinically warranted. Furthermore, many clinical subspecialties in addition to pediatrics and medical imaging offer supportive diagnostic information that must be incorporated in the overall analysis of a case. The ophthalmologist, critical care specialist, emergency medicine physician, trauma surgeon, and neurosurgeon play vital roles in the clinical assessment. Investigative collaboration requires a close relationship with biomechanical engineering, law enforcement, Child Protective Services (CPS), and forensic pathology. Child abuse is unique compared with other diagnostic processes because there is no single or combined set of clinical indicators that proves a child has been abused. In fact, there are many medical conditions that can mimic some or all of the indicators seen in child abuse. Laboratory testing and clinical evaluation is essential in the identification of confounding medical conditions. However, in the context of a traumatic brain injury (TBI) in an infant or young child who, in the absence of other medical factors, does not have a sufficient history of trauma to account for the injuries, AHT leads the differential diagnosis.
Terminology and history of AHT
In 1946, Caffey described a series of six cases of chronic subdural hematoma (SDH) in infants with “complications” of long bone fractures. There was no history of trauma provided by any of the families and there was no evidence of bone disease in any child. The origin of these combined findings seemed obscure. One child was reported to be “clearly unwanted” by the parents, and treatment of the child was questioned. Caffey was not certain that the same traumatic forces were the cause of both the fractures and SDH because the fractures occurred weeks and months after the hemorrhage in several of the cases. Regardless, Caffey recommended investigation of SDH when long bone fractures were identified, and routine roentgen examination of the long bones when SDH was diagnosed (1). A case study published in 1950 credits Caffey’s paper in aiding the diagnosis of an infant with SDH after the discovery of multiple healing fractures (2). By 1965, Caffey, in his Howland Award Lecture, exhorted physicians to consider trauma as the cause of a child’s fractures, even without a history of trauma. However, he cautioned against overzealous accusations, as the parents may not know the trauma even occurred. He also commented about the range of injuries from accidental trauma and stated that severe trauma may be a matter of “pure chance.” As an example, he described a case of an 11-story fall where the child only suffered a fibular fracture and compared it to a case of a child who sustained a SDH from a much shorter fall (3). In continuing his observations on infant abuse, fractures, and SDH, Caffey coined the term parent–infant traumatic stress syndrome or PITS (also called the Caffey–Kempe syndrome) (4). Caffey’s journey to discover the underlying cause of the unexplained fractures, specifically metaphyseal injuries, and SDH continued. He described an “infant nurse” who allegedly killed three infants in her care, two being sisters, and permanently injured another infant. The nurse admitted to giving at least one of the infants “a good shaking,” and at autopsy the child had TBI. In a published address, he further expanded upon the case and described 2 additional infants that were “maimed” and 10 others that had been seriously injured by the same nurse (5). He provided 27 clinical examples of infants that were shaken and noted that two of those cases, plus several others reported in the literature, had found retinal hemorrhages (RHs) in association with the shaking. It was at this time, over 40 years ago today, that he called for a massive nationwide campaign to alert everyone to the dangers of shaking infants.
In 1974, Caffey coined the term “whiplash shaken infant syndrome,” to describe those children suffering SDH, RHs, and neurologic injury. Specific skeletal injuries, such as metaphyseal fractures or “traumatic involucrum” and “traction lesions” of the periosteum of long bones, often accompanied the brain and retinal injuries (6). Caffey often referred to the works of Guthkelch and Silverman, as well as Ommaya and Yarnell’s early biomechanical studies on adult primates (7). Guthkelch proposed that, although SDH was most often traumatic, in infants, the cause may not always be attributed to head impact, but may also be caused by acceleration/deceleration of the head (8). These authors initially exhibited reticence at suggesting parents or caregivers injured infants, whether intentionally, innocently, or in a moment of anger or stress. However, the evidence became overwhelming when SDH, whether chronic or acute, was accompanied by other signs of injury, particularly skeletal trauma and RHs. Even today, their observations provide an astute analysis that in the absence of a traumatic history or confounding medical conditions, TBI sustained by a young infant is likely to be inflicted by a parent or caregiver.
In 2009, the American Academy of Pediatrics (AAP) Committee on Child Abuse and Neglect recommended that the term “abusive head trauma” be used because it more appropriately described traumatic injuries to the brain as a result of abuse (9). The term “shaken baby syndrome” (SBS) implies a single mechanism of head trauma, whereas AHT is more encompassing of the multiple mechanisms of abuse such as crushing head injury, shaking, shaking with impact, impact alone, or strangulation. Furthermore, the term “syndrome” suggests that each case must have a well-defined constellation of findings. Historically, a triad of symptoms, SDH, cerebral edema, and RH suggested a diagnosis of AHT. However, each clinical case differs from the next in both obvious and subtle ways. A child with SDH, multiple contusions, and fractures, but without RHs may clearly be abused but would not precisely fit the simple diagnosis of SBS. However, it should be noted that the AAP Committee did not suggest that the term “shaken baby syndrome” be abandoned because it is still a well-recognized entity for public education and prevention programs aimed to reduce the incidence of AHT.
Epidemiology
From the population-based studies by Keenan and colleagues, we are painfully reminded that inflicted TBI is the leading cause of death in infants and young children (10, 11). Keenan et al. prospectively collected data on serious and fatal pediatric TBIs from nine Pediatric intensive care units in North Carolina. They reported an incidence of 17 per 100,000 person-years in all children under the age of 2 years (11). In infants less than 12 months the rate was markedly higher at 29.7 per 100,000 person-years. These incidences of AHT are strikingly similar to those reported in other regions of the world. Barlow and Minns prospectively evaluated the annual incidence and demographics of nonaccidental head injury in Scotland and found this incidence to be 24.6 per 100,000 in children less than 1 year of age (12). Kelly and Farrant reported the annual incidence of inflicted infantile SDH in New Zealand to be between 14.7 and 19.6 per 100,000 in infants under 1 year of age (13). Despite differences in methodology, and minor differences in definition, the message is clear: AHT is a global problem that requires clinical and biomechanical data to be shared locally and globally in order to increase the efficiency and speed with which we can develop and implement prevention, detection, and treatment programs for AHT in children.
Clinical diagnosis of AHT
When compared to children with known accidental head injuries, children diagnosed with AHT are more likely to lack a reasonable history of injury, have intracranial hemorrhage, present with a lower Glasgow Coma Score (GCS), have more severe outcomes, and have RHs (14–17). However, infants and young children may present with less severe head trauma that is not clinically obvious. Jenny et al. reviewed 173 cases of AHT that had been missed when the child first presented to medical providers (18). These infants often presented with symptoms of vomiting, irritability, or lethargy. They received diagnoses of acute gastroenteritis or colic, or possible sepsis. Many children who were missed would not have met the criteria for cranial imaging based upon their symptoms and physical examination. This study emphasizes the difficulty in making a diagnosis of AHT in young infants whose clinical presentation may account for a number of differential diagnoses. One key factor in infants with less severe injuries or nonspecific symptoms, however, is bruising. Feldman compared bruising patterns in infants who had not yet become mobile to those in mobile infants and toddlers. Bruising in the young pre-mobile infants was exceedingly rare (19). Maguire et al.’s systematic review of the literature identified patterns of bruising in abused and nonabused children, and found that bruising in nonmobile infants was highly suggestive of abuse (20). Combined with Jenny’s study, these reports suggest that young infants with bruising, with or without nonspecific symptoms such as vomiting, irritability, and lethargy, are at significant risk for having suffered AHT and an appropriate evaluation should be pursued. Clinical indicators, such as bruising, become critically important in young infants as many of the infants who were missed in Jenny’s study went on to suffer from more severe abusive injuries, including five deaths that could have been prevented by earlier diagnoses (18).
A detailed and specific history by an experienced clinician at the earliest possible time during the patient’s hospitalization is critical to the diagnosis of AHT. Exhaustive attention must be paid to the prenatal and birth history, growth and development, temperament, illnesses, other injuries, neurodevelopmental parameters, and family and social history. Specific details of the traumatic event are also necessary. For example, if a fall is proposed, an estimate of height, surface, and other environmental factors is important. This should be followed by a detailed chronology of the child’s symptoms and a retrospective review of several days to a week or more of feeding patterns, general activity, and responsiveness. Noting different caregivers and other individuals who had contact with the child provides additional corroborative observations that support or refute the primary caregiver’s explanation. A review of all primary care records, and reconstruction of not only general growth parameters, but also a head-growth velocity curve provides additional data depending on the nature of the head injury.
The child who presents in extremis, with seizures, loss of consciousness, or apnea, elicits more straightforward emergency medicine and diagnostic procedures. Cranial imaging, specifically initial nonenhanced head computed tomography (CT) is one of the first diagnostic tests in any acute presentation following stabilization of the patient. Intracranial hemorrhages such as subdural, subarachnoid, epidural, and cerebral parenchymal hematoma raise concern over a traumatic event. Careful clinical assessment may provide a reasonable explanation for this child’s presentation. However, trauma is the leading cause of SDH in infants whether accidental or inflicted (21–23). This does not imply that trauma is the only cause of SDH in infancy and a differential diagnostic list of nontraumatic causes of infant SDH is listed in Chapter 18 (see Table 18.8). SDHs are reported in up to 46% of term newborns, most following vaginal delivery, and many of these are asymptomatic (24). Medical causes of SDH in infancy include infectious, metabolic, neoplastic, and hematologic conditions. Most of these conditions become obvious during the medical assessment process. In an infant who presents with SDH or any findings where the differential diagnosis includes abuse, a careful clinical, laboratory, and medical imaging approach is needed (25–27).
The presence or absence of neck injuries has never been proposed to be integral in making a diagnosis of AHT. Neck injuries, although far from universal in AHT, may be observed in imaging studies and autopsy, and can provide additional clinical information regarding the mechanisms of injury (28, 29). Although victims of AHT are predominately infants (<12 months old), children up to 8 years of age have also been reported to present with similar findings and outcomes (30).
Biomechanical foundations for AHT
Biomechanics is the study of forces applied to the human body, and the human body’s response to those forces. It can be studied on a macroscopic scale (head impact resulting in skull fracture) or on a microscopic scale (individual axonal stretching during head rotation). Biomechanics of head injury was first investigated in the late 1960s and has played an important role in understanding mechanisms of TBI. In the 1980s, researchers began turning their attention specifically to pediatric head injury. It was initially assumed that children were miniature adults and the mechanical response of the head to a force could be scaled according to head mass. However, it has since been shown that children are biomechanically unique from adults. The mechanical response of the brain, skull, and neck to trauma changes nonlinearly throughout early development (31–36). Injury thresholds and the ability to recover from an injury are also highly dependent on the developmental age of the child (37, 38). It is often challenging to quantify these developmental differences in children because of the small number of human pediatric specimens available for research. Instead, biomechanical engineers must rely on age-related data from other sources such as animals and cadavers, and then relate the findings to children using computational modeling.
Animal experiments allow us to test complex hypotheses regarding the age-dependent biologic response to trauma in a controlled environment. Specific mechanisms of trauma such as pure head rotation, impact contusion, or pressure wave propagation can be isolated to identify brain injury specific to one mechanism or shared by multiple mechanisms. For example, rapid head rotation may result in diffuse traumatic axonal injury and widespread subdural and subarachnoid hemorrhage (39), while focal impact contusion typically results in local SDH and neuronal loss at the impact site (38). Age-related injury thresholds are specific to the mechanism of injury. Ibrahim et al. (37) reported a lower threshold of axonal injury in young animals when experiencing a pure head rotation compared to older animals. However, Missios et al. found the opposite to be true for studies involving focal impact contusions (38). Translating injury tolerances from animals to humans is challenging, but what we have discovered thus far is that the severity of head injury in children is dependent on: (1) the age of the child (38); (2) the mechanism of head trauma (37, 38); (3) the length of time after the trauma (40); (4) the direction of head rotation and/or the location of head impact (39); (5) the number of insults to the head (41); and (6) the period of time between those insults (40). There is still much that is unknown, specifically about AHT, but animal experiments will continue to be necessary to improve our understanding of the biomechanics of head trauma in children.
Cadaveric testing of whole bodies, organs or small pieces of organs can provide significant insight into the human mechanical response to a force. One factor that must be carefully considered with cadaveric testing, however, is the degradation of tissue post mortem. The mechanical response of bone is maintained fairly well if it is frozen after death and then thawed for testing (42–44). However, some studies have shown that if frozen too long (>100 days), the properties of bone change and it is no longer a valid representation of in vivo responses (45). Softer tissue degrades much faster than bone. For example, brain tissue maintains its mechanical properties for up to three hours post mortem (46) and loses structural integrity after six hours (47, 48). For this reason, most pediatric whole-body or organ cadaveric tests investigating pediatric head trauma typically focus on bony structures such as the skull (see Chapter 17), and the mechanical response of soft tissues is commonly identified through material property tests on specimens obtained from surgery. Prange and Margulies (35, 49) tested cerebral cortex excised from temporal lobectomy procedures performed on several adults and one five-year-old child. From these specimens, they found that the stiffness in shear (i.e., shear modulus) of the five-year-old brain was greater than the adult brain when undergoing large deformations. This means that more force per unit area was required to deform the pediatric brain compared to that of the adult. Although this finding is based on a single human specimen, similar relationships have been reported in pig and rat brains (35, 50). Note, however, that while it may be more difficult to deform the pediatric brain, less deformation may be required to injure the pediatric brain during head rotation compared to adults (37). To obtain a complete picture of brain injury all regions of the brain must be considered. Shear tests from cerebral cortex, corona radiata, thalamus, and corpus callosum in porcine brains have demonstrated a mechanical heterogeneity within the brain (35, 51). Peripheral regions of the brain (cerebral cortex and corona radiata) are significantly stiffer than central regions of the brain (thalamus and corpus callosum), and gray matter is significantly stiffer than white matter. Adjacent tissues with a mismatch in mechanical properties can lead to a higher probability of injury at the interface depending on the mechanism of the trauma. For example, gliding contusions from rotational head injury may occur because the cerebral cortex is stiffer than the corona radiata, resulting in relative motion between the two structures. This injury would not typically occur following a focal impact. Mechanical tests performed on human pediatric dura (52), skull (31, 33, 34, 36), and cranial suture (31) have provided similar insight into mechanisms and thresholds for injury to those structures of the head. The biomechanical studies that are still missing, however, are investigations of changes in the structure and mechanical properties of pediatric cerebral vasculature, and the mechanical characterization of the interaction between the cerebral vasculature, dura, arachnoid, and pia. These studies will be necessary before we can begin to understand mechanisms of SDH.
One ultimate goal of biomechanics is often to relate injury to the instigating force. This is particularly challenging in pediatric head trauma because every inflicted and noninflicted trauma is unique. Furthermore, a reliable and detailed history of the traumatic event is seldom provided for infants. To overcome this obstacle, finite element (FE) modeling can be used to investigate changes in mechanical response with varying environmental parameters (e.g., fall height, impact surface, body impact site) and anatomical features (e.g., enlarged subarachnoid space [SAS], state of brain maturity, and development of the craniocervical junction). Roth et al (53) used FE analysis to evaluate the sensitivity of injury predictions to errors in estimating fall height and impact location. They concluded that a 30% inaccurate estimate of fall height resulted in a 15% error in FE model predictions for occipital and parietal impacts and a 19% error for frontal impacts. Inaccuracies in the described location of skull impact within a single cranial bone resulted in 8% error for frontal impact, 13% error for occipital impact, and 25% error for parietal impact. It is clear from this study that large inaccuracies in the history will affect the ability of FE analysis to be used in injury prediction. Furthermore, just as every trauma is unique, the anatomy of every child is unique. Coats et al. investigated the effect of suture width on predictions of skull fracture in a 1½-month-old infant FE model (54). Small differences in suture width (~2–3 mm) did not significantly affect predictions of injury, but large increases in suture width (~7–8 mm) resulted in 43.2% decrease in the prediction of skull fracture. Suture thickness did not significantly alter predictions of skull fracture. Therefore, small variations in suture will not likely alter the mechanical response of the head, but an infant with unusually large sutures will have a drastically different mechanical response to impact. Raul et al. investigated the effect of a large SAS on predictions of strain (deformation) in bridging veins spanning the SAS (55). Using a simplified model of vasculature and brain–skull interaction, the authors significantly increased the region between the skull and brain from 2 to 8 mm, and connected this space with linear elastic connectors representing bridging veins. A shaking motion was simulated in the model. The strain in the bridging veins was reduced by 76% at the vertex and 38% in the occipital region of the head when the space was enlarged. Strain was calculated as the stretch of the bridging vein divided by the original length. Therefore, in the enlarged model, the original length of the bridging veins increased without a respective increase in the stretch. This resulted in the lower bridging vein strain. The biomechanics of bridging veins and their interaction with the dura, arachnoid, and pia is not well understood and this FE model is likely a simplification of the actual structures involved in SDH.
One notable attribute of FE modeling is that it will always provide its users with an answer, regardless of whether it is the correct answer. Therefore, it is very important for all FE models to be critically evaluated before they can be trusted. The parameters used to create the model (material properties, geometry, tissue interactions, load conditions) need to be carefully reviewed to determine whether they are realistic, or whether simplifications are justifiable for the scenario being modeled. Furthermore, if the objective of the model is to predict injury, then it must be thoroughly validated against experimental or clinical data before those predictions can be trusted.
These are just some of the many biomechanical tools used to investigate and further our understanding of pediatric head trauma. Specific studies using them are incorporated throughout the next few sections as we discuss the state of clinical and biomechanical knowledge in specific subtopics of AHT including SBS, RH, and noninflicted trauma. A more in-depth discussion on the importance of biomechanics in understanding pediatric head trauma can be found in a recent contribution by Margulies and Coats (56).
Shaking as a mechanism of intracranial injury
Clinical data
Perpetrator confessions and case studies provide support for shaking alone as a mechanism of AHT (57). In many cases of abuse, caregivers inform clinicians or police interrogators how they caused an abusive injury (58–61). Such information, although limited, does provide an insight into the mechanisms of AHT. A discussion of whether confessions are true or perhaps coerced by overzealous interrogators is merited (62–65). Typically, however, these recounts are informative and provide unique parallels. Starling et al. compared 81 cases with a perpetrator admission of AHT to 90 cases with no admission of abuse (66). Fifty-five of the 81 perpetrators admitted to shaking the child, 44 without impact. Even when the perpetrator described impact to the child, not all of the children had evidence of impact from clinical examination. None of the 171 children in this study were described as acting normally after the event. Adamsbaum et al. examined forensic evidence from 112 cases referred for AHT (67). In 29 cases there were confessions and in 83 there were not. When AHT was diagnosed, there was no difference in the clinical and radiologic presentation between those whose caregiver confessed and those who did not. This was similar to Starling’s findings. What was remarkable was not only the violence that the perpetrators described when they shook the child, but also the repetitive nature of the abuse. Most often a child is shaken because of crying. Shaking an infant stops the crying. Therefore, the adult continues to use shaking as a means to stop the infant crying. Another study reviewed seven cases of confessions during police interrogation (68). Five perpetrators confessed to shaking, two of them also indicated impact. The paper describes in detailed narrative form what was done to the infant. In most cases the initiating event was crying. A comparison of AHT inflicted by male and female perpetrators reported that 14 of 15 males described or demonstrated shaking their victims (69) whereas only 3 of 17 alleged female perpetrators confessed. Male perpetrators were more likely to fatally injure a child and overall caused more serious injuries, perhaps because male perpetrators are on average larger and stronger than female perpetrators and can generate greater forces. Taken as a whole, the consistency of perpetrator confession reported in the literature and the injuries seen in these children provides tremendous supportive data for shaking as one mechanism of injury in AHT (1–5, 8). Others have taken a more skeptical view of prevailing accumulated medical knowledge regarding shaking and AHT (70, 71).
Biomechanical data
Very little data exists regarding the biomechanics of shaking. This has led to substantial disagreement regarding the potential for injury from shaking. What we do know from biomechanical experiments to date is that the rotational velocity experienced by the infant head during shaking is about the same as what might occur from a head impact onto concrete after a 1-ft fall (72). However, the duration of a head impact onto concrete is much shorter than the duration of a chin impact to the chest during shaking. This means that the deceleration of the head during shaking is approximately 10 times lower than the deceleration from a 1-ft fall onto concrete. To understand the difference between velocity and deceleration, imagine a fist hitting a padded wall versus a concrete wall with the same initial velocity. The fist stops much more abruptly against the concrete wall (higher deceleration) and is more likely to result in internal injury than when it hits a padded wall. The same is true for head injury. Larger decelerations result in higher risks of injury. Therefore, based on head deceleration alone, there is a much lower risk of brain injury from shaking than from a 1-ft fall onto concrete. However, shaking is unique in that this low level acceleration/deceleration is being applied multiple times in a row instead of just once as in a fall. Animal studies focused on investigating the effect of repetitive head injury have primarily been performed in rodents. Smith et al. developed a shaking model of brain injury using male Sprague Dawley rats (73). P6 rats were attached to a pneumatic shaker that shook rats 50 times at 3.3 Hz for 6-second rest intervals in between. Cortical hydroxyl radicals, vitamin E levels, and percentage area with the cortical hemorrhaging were significantly higher in the shaken rats compared with shams. Of note, however, is that the rats were subjected to hypoxic conditions before and during the injury. In another model of shaking (74), P8 Swiss mouse pups were shaken for 15 seconds on a horizontal rotation shaker at a frequency of 900 cycles per minute (15 Hz). White matter hemorrhagic lesions were present at P13 and cysts developed between P15 and P31. The small lissencephalic brains of rodents with little gray/white matter differentiation make it challenging to translate this data to rotational head injury in children. Furthermore, anthropomorphic surrogate studies of shaking typically report shaking frequencies of 3–4 Hz (72, 75), which is much lower than 15 Hz used in the aforementioned study. One of the few shaking studies performed in a larger animal model was reported by Finnie et al. (76). Seven 7–10-day-old anesthetized lambs were manually shaken 10 times for 30-second durations over a period of 30 minutes. There was a neuronal cell death response to the shaking, but the authors acknowledge that this was likely a nonspecific acute response to the trauma. Axonal injury was minimal and only 2 of the 7 animals had a small 1 × 1 cm SDH. In a subsequent study, Finnie et al. performed additional experiments in lambs of lower body mass. Three of these animals died at 2, 3, and 5 hours after the final shaking episode. Because the shaking was performed manually and there was no instrumentation, it is unknown what forces were produced from this experiment, how the brain mass relates to the human infant brain mass, or how relevant the forces are to an adult shaking an infant (77).
More recently, Coats et al. explored the effect of repetitive head rotations compared with a single head rotation on TBI in immature piglets. They found that at low velocities similar to those measured during shaking, repetitive head rotation resulted in only slightly more injury than a single head rotation. Neither the single nor the multiple low velocity head rotations resulted in the magnitude of injuries reported clinically. Interestingly, there did appear to be a delayed response of axonal injury and extra-axial hemorrhage from the multiple head rotation that was not seen in the single head rotation (78). Even with progress in improving our animal models of shaking, the current biomechanical data on the topic is not enough to experimentally prove or disprove the ability for shaking to cause TBI. More work needs to be done to investigate neck injury and mechanics from shaking, and the timing and progression of injury from repetitive head rotation.
Retinal hemorrhage
Clinical data
The optic nerves and eyes represent ventral projections of the central nervous system, thus warranting a discussion of RH under the topic of abusive head injury (79). RH associated with possible inflicted head trauma was first described by Caffey (5), but there have been multiple observations and clinical studies since. From these studies, RH has been reported in 0–20% of accidental trauma (80–85), and 37–92% of AHT (80, 85–91). Improved assessment of RH has led to an increased distinction between the type and distribution of RH in accidental trauma and AHT. Minor accidental trauma may result in a sparse distribution of RH or RH isolated to the posterior pole of the globe. Severe RHs are more typically found in cases of AHT (22, 92, 93). Severe RHs can be characterized by their presence in multiple retinal layers and extension to the ora serrata. Traumatic retinoschisis or splitting of retinal layers with blood when seen in the context of SDH also implies very severe head trauma and has a strong association to AHT (94, 95). The presence of extensive RH, however, should not confound the diagnosis of AHT when they occur in the context of severe accidental head injury. Children who suffer crushing head injuries when large heavy objects fall on their heads have been described to have severe retinal injury (81). One to three percent of children in motor vehicle crashes can have extensive RH (82, 96). A 2-year-old child in Australia who fatally fell 11 m onto concrete with resulting multiple skull fractures and mass occupying SDH was also reported to have RH and retinoschisis that appeared similar to typical intraocular findings of AHT (97). An investigation supported the accidental nature of this injury. Other medical conditions in children that may be associated with RHs are meningitis, metabolic disorders, hemorrhage diseases with coagulopathy, and neoplasms (93). Adams and colleagues have reported their observations of the appearance and location of RHs in critically ill children (98). Goldman and colleagues described RHs associated with severe cough (99). Increased intracranial pressure has been associated with RH in the perineural area, and may be associated with venous congestion (100). These medical conditions, however, are not readily confused with AHT in the clinical context. A thorough assessment of an infant or young child for AHT should include a dilated retinal examination with either careful descriptive diagrams or retinal photography. In fatal cases, specialized techniques for removal of the globe and pathologic evaluation by forensically trained pathologists and ophthalmologic pathologists is essential (101). An ophthalmologist with training in the evaluation of pediatric patients should be part of the diagnostic team in child abuse assessments and can provide differential diagnoses and clinical perspective in etiology of retinal findings.
Biomechanical data
To date, many theories exist regarding the mechanism of traumatic RHs, but none have been scientifically proven. The most referenced theory is that adhesion at the vitreoretinal interface causes the vitreous to pull on the retina during rapid head rotation damaging the retinal vasculature. Several of the animal shaking studies mentioned earlier also investigated ocular injury. Bonnier et al. (74) reported RHs in 30% of the rodents shaken at 15 Hz. Finnie et al. report a few RHs in 2 of the 7 shaken lambs (29%) (76). None of the pig eyes in Coats et al. had RH (78).
In a preliminary investigation to identify the mechanism of RHs, Coats and colleagues performed histology on immature porcine eyes after they experienced rapid (117–226 rad/s) head rotations. Ocular hemorrhage was found in 73% of the eyes with 51% being bilateral. The majority of the hemorrhages were found in ciliary body (70%) and only a small percentage (11%) had RH. All RHs were located in the periphery and limited to one or two in total (102). While the load mechanism investigated was not repetitive, similar to shaking, it was interesting that all injuries occurred near the vitreous base of the eye, which has qualitatively been determined to have greater vitreoretinal adhesion in the pig (103). This supports, but does not prove, the theory of vitreoretinal traction. Other theories that bear exploring are rapidly increasing intraocular pressure as a result of intracranial pressure changes and repetitive vascular occlusion from compression of globe against orbit during shaking.
In addition to animal studies, there have been two FE models used to investigate the biomechanics of the eye from repetitive loading. Hans et al. investigated differences in retinal force between shaking and a 45-cm fall (104). They report that retinal force was 50 times higher during shaking than compared to the head impact of a fall. Unfortunately, the head motion of the fall was simplified as being translational (no rotation of the head), which is not a realistic representation of a fall (105). Furthermore, there are currently no material properties available in the literature for pediatric ocular tissues. The model by Hans et al. utilized adult material properties and the values reported by the model are likely not reliable for predictions of injury in the pediatric eye. Because pediatric properties are unknown, Rangarajan et al. performed a parametric FE study to identify the importance of vitreous and fat material properties on retinal stress during repetitive head rotation (106). Not surprisingly, the retinal stress in the model was highly dependent on the material properties of vitreous. To date, vitreous has only been quantitatively characterized in adult eyes, but qualitative studies on changes in vitreous structure with age strongly suggest that pediatric eyes will have a very different mechanical response than adult eyes (107). Complete characterization of the changes in pediatric ocular tissues needs to be completed before FE modeling can fully be used to investigate mechanisms of RH in AHT.
Accidental or noninflicted trauma
Clinical data
A history of a short fall is often provided as an explanation by a caregiver for a child’s serious or fatal injury. Therefore an analysis of fall data is imperative to evaluate the risk of such injuries from short falls. Helfer et al. in 1977 tried to identify the types of injuries suffered when children fell out of bed (108). They used two types of data, questionnaires from parents whose children (<5 years old) fell from a bed, and hospital-incident data acquired when children fell in hospitals. In 161 parent-reported falls, 37 injuries were of a nonserious nature (lumps, bumps, and bruises). The six “serious” injuries that were reported were skull fractures without serious head trauma, a humerus fracture, and three fractured clavicles. The hospital incident data included 85 reports of children who fell approximately 90 cm. A 1983 study by Barlow reviewed social and medical data on 61 children admitted over 10 years with falls from 1 or more stories in New York City (109). Seventy-seven percent of the children survived. Of the children who fell three stories or less, all survived. Fifty percent mortality occurred from falls between the fifth and sixth floors. Studies of children who are witnessed to fall short distances have demonstrated the benign nature of those falls. Nimityongskul and Anderson reported on 76 children from birth to 16 years of age that were reported to have fallen out of a bed, crib, or chair while in the hospital (110). Seventy-five percent of the incidents occurred in children from birth to five years of age. The height of falls ranged from 1 to 3 ft. Most of the injuries were minor (scalp hematoma and facial lacerations). This study indicates that severe head, neck, spine, and extremity injuries are extremely rare when children fall out of hospital beds. They concluded that child abuse should be investigated when a child is seen with a severe injury from a reported “fall at home.” Chadwick et al. took a slightly different approach when evaluating falls in children (111). By comparing the “history” of the height of the fall provided by a caregiver with the severity of the injury, they found that 7 deaths occurred in 100 children who were reported to have fallen 4 ft or less. One death occurred in 117 children who fell 10–45 ft. The seven children who died reportedly following short falls all had other factors in their cases that suggested false histories. This study suggested that when fatal injuries occur with a history of a fall of less than 4 ft, that history is often incorrect. Another study from Montreal reviewed 64 children who fell greater than 10 ft over a 3-year period (112). Thirty-six percent of the patients were less than four years old. Fifty patients fell from 20 ft or less; 14 fell from greater than 20 ft. In falls less than 20 ft, significant injuries were uncommon and there were no deaths. Head injuries consisted of concussions, skull fractures, and other injuries involving the extremities. Multisystem injuries were more common in the children who fell more than 20 ft. The only death occurred following a 5th story fall (50 ft) similar to the Barlow study. An Austrian study of 103 children who fell from high chairs, reported that of the children who suffered head injuries, 16 had linear skull fractures, 14 had concussions, and 71 had contusions or lacerations of the face or head (113). Only four infants were taken to medical care for minor injuries. Contrasting studies suggest infants may be seriously injured or die from short falls. Plunkett reviewed Consumer Product Safety Commission data on 75,000 falls involving playground equipment (114). There were 18 deaths documented. In the 8 fall deaths in children under 3 years of age, the youngest child was 12 months. Three of these were falls from swings, one from a seesaw, three from platforms, and one from a ladder. The height of the fall was measured from the lowest point in the fall rather than the distance from the head to the ground. Three of these children had complex calvarial fractures; the other five were reported to have died from cerebral edema, and herniation, only three with acute SDH. One of the three-year-olds suffered from thrombocytopenia-absent radius syndrome and may have been prone to bleed. Spivack, in a letter to the editor regarding this paper, suggests that falls from swings are more complex than short vertical falls, and clarifies several flaws in Plunkett’s analysis (115). Some of the children had RHs, but not one had formal evaluations by an ophthalmologist (116). Chadwick et al. calculated the annual risk of deaths from short falls in children (117). Using a variety of sources, including the California Epidemiology and Prevention for Injury Control Branch, 6 short-fall deaths were reported in a population of 2.5 million children. This study estimated that, although not impossible, the risk of death from a short fall (defined as <1.5 m) was less than 0.48 deaths per 1 million young children per year. These studies point to the importance of careful clinical analysis of each short fall death or serious injury. A thorough medical and social services evaluation must be performed in any case where a child suffers a serious or fatal intracranial injury (ICI) from a short fall. In a very small number of cases, this type of evaluation may help prove the history of a short fall to be true. Additional injuries, such as fractures, RHs, cutaneous injuries, and previous abuse history (whether the patient has been previously abused or the caregiver has a history of abusing children), must be part of the process of assessment.
Biomechanical data
Low height falls are the most common history provided by caretakers suspected of child abuse and are the most common noninflicted trauma in children (118). Because of this, the majority of the biomechanical literature on noninflicted trauma deals with identifying probabilities of injury from low height falls in order to better recognize injuries that are more likely to fall under the auspices of AHT. Epidemiologic studies investigating injuries from low height falls provide useful insight into the trends of noninflicted injuries, but because every fall is unique and complex it is difficult to use the data to assess injuries on a case-by-case basis. Biomechanics is much better suited to evaluate all the complexities of a fall and estimate injury. The caveat, however, is that validation of these estimates is difficult because falls are seldom witnessed or described in enough detail. In lieu of these challenges, the majority of biomechanical fall research has focused on making comparisons between the forces involved in inflicted and noninflicted trauma, and identifying fall characteristics that are important to predicting injury in a fall.
One of the earliest biomechanical studies investigating the biomechanics of a fall was by Prange et al. (72). They created a simplified infant surrogate consisting of a torso, head, and hinged neck. The hinged neck was selected to maximize anterior–posterior motion and evaluate a worst-case scenario while measuring head angular acceleration during occipital impact following 1–3-ft falls onto mattress, carpet, and concrete. Not surprisingly, falls from higher heights onto harder surfaces resulted in higher angular velocities and angular accelerations, and would translate to a higher risk for injury. To increase the biofidelity of the surrogate, Coats and Margulies (105) added cranial sutures to the skull and selected a multidirectional neck based on the stiffness of a single C3–5 segment from a 23-day-old infant cadaver. They repeated the occipital impact falls performed by Prange. The addition of the more deformable skull and multidirectional neck resulted in angular accelerations and velocities that were 36–88% lower than the Prange surrogate. This finding is likely attributed to the much larger deformations of the skull, which dissipate some of the impact energy. Furthermore, the motion of the head was no longer confined to a single plane and was dominated by both sagittal and horizontal head rotation. Injuries associated with these surrogate studies cannot be estimated because of the lack of age-appropriate experimental data. However, a recent study by Eucker et al. stressed the importance of identifying head rotation direction when predicting injury (39). In the study, 36 piglets underwent similar magnitudes of either sagittal, coronal, or horizontal head rotation. Sagittal head rotation resulted in more severe intracranial injuries than the other head rotation directions. Horizontal head rotation also resulted in significant pathology, but coronal head rotation produced only small amounts of ICI. This suggests that during falls and other noninflicted or inflicted trauma, the location of head impact or direction of head rotation will significantly influence the severity of the head injury.
Thompson et al. used a commercially available 12-month-old surrogate (CRABI) to identify feet-first fall characteristics that result in higher risks of injury (119). Similar to the aforementioned surrogate studies, the floor surface significantly affected the angular acceleration of the head. However, in contrast to the earlier studies Thompson et al. report that lower height falls onto linoleum and wood resulted in higher angular accelerations than higher height falls. This is because the kinematics of a feet-first fall are very different from those of a head-first fall. For higher heights, the surrogate feet impacted the floor and the legs buckled. This absorbed some of the energy of the fall prior to the torso falling backward and the head impacting. In the lowest height fall, the legs did not buckle and the body of the surrogate pivoted backward about the heel of the foot. This does not mean, however, that a lower height fall is worse than a higher height fall. A subsequent study by Thompson et al. investigated fall characteristics and injury from 79 clinical cases (120). Fifty-three percent of their investigation was based on caregiver interviews and 47% were based on interviews and scene investigations. Fall height, floor surface, subfloor material, pre-fall position, post-fall position, and the child’s body mass index (BMI) were investigated in each case and correlated to the highest Abbreviated Injury Scale (AIS) score of the child. Fall height had the largest correlation with AIS score, with higher heights correlating to higher scores. Floor surface was not found to significantly correlate to the AIS score, but this may be due to large variations in floor and subfloor surfaces among the cases. One interesting finding of the study was that a lower BMI significantly correlated with a higher AIS score. Because the locations of the injuries were not statistically evaluated, it is unclear whether higher fall heights and decreased BMI were more associated with injury to the head or to other locations of the body.
All the aforementioned surrogate studies have focused on infants below 12 months of age. To investigate the biomechanical differences between toddlers and infants, Ibrahim and Margulies (121) created an 18-month-old toddler surrogate and repeated several of the experiments performed by Coats and Margulies (105). The toddler surrogate had a thicker fused skull, stiffer neck, and heavier mass than the infant surrogate. As might be expected, angular acceleration and impact force in the toddler surrogate were on average 1.6 and 5.0 times greater, respectively, than in the infant surrogate. To better understand how this might relate to injury, Ibrahim and colleagues investigated clinical cases of low height falls in infants and toddlers (122, 123). Toddlers were found to have a higher incidence of altered mental status as defined by lethargy, sluggishness, unexplained irritability, or loss of consciousness (65% vs. 31%), but a lower occurrence of skull fracture (23% vs. 73%). Combining both studies, Ibrahim et al. concluded that larger head angular accelerations measured from the surrogate experiments concur with a higher incidence of altered mental status. However, the greater incidence of altered mental status in toddlers than infants could also be attributed to increased ease with assessing those parameters in an older child. They also concluded that although the head impact force in the toddler was larger, the toddler skull is thicker than the infant skull and able to withstand more force before fracture.
Noninflicted trauma in children is complex and biomechanics will be required to truly evaluate the potential for injury on a case-by-case basis. However, true success in understanding head trauma in children will require a strong collaboration between clinicians and biomechanical engineers. Clinicians are on the front line and are the gatekeepers of the data used to create biomechanical models of injury. Collecting detailed histories that include particulars such as fall height, floor surface, subfloor type, pre-fall position of the child, post-fall position of the child, and initial impact location on the body will be vital to creating and validating biomechanical predictions of noninflicted traumatic injury. Once validated, however, these models can be extremely valuable for exploring and clarifying the multifaceted characteristics of AHT.
Cost to society
All forms of TBI impact the fabric of a family and society. Injuries to the brain and central nervous system have the potential to permanently impair an individual’s cognitive, motor, and emotional functions (124, 125), and require more intensive interventions and longer stays. Tremendous resources are required for rehabilitation and subsequent habilitation as the individual’s ability to fully function as a member of a family and society, and as a social and economic contributor is compromised. The cost to families is immeasurable as they struggle to cope with a member whose life has changed, and whose needs have significantly expanded.
AHT, a condition that primarily affects the youngest and most vulnerable, leaves up to half of its victims with permanent impairments. One study suggested that the cost of each child who suffers from AHT is upwards of $8 million for lifetime costs. In acute care hospital costs, those children diagnosed with AHT have significantly higher hospital charges compared to nonabused children (125). They tend to be more symptomatic on admission, have higher frequencies of cerebral edema, anoxic/ischemic injury and worse overall outcomes as compared to children with noninflicted injuries (17). This information correlates with a study by Ewing-Cobbs et al. that also demonstrates worse neurologic outcomes of AHT compared with accidental head injuries (126). Not only do children with AHT have significant brain injury, but the delay in seeking care following the abuse is more common and compounds the brain injuries. The apnea that often follows acute head trauma in infants can result in hypoxic ischemic injury. All of these factors lead to poorer outcomes overall. Therefore, permanent lifelong disabilities and subsequent economic costs associated with those conditions are significant (127, 128).
References
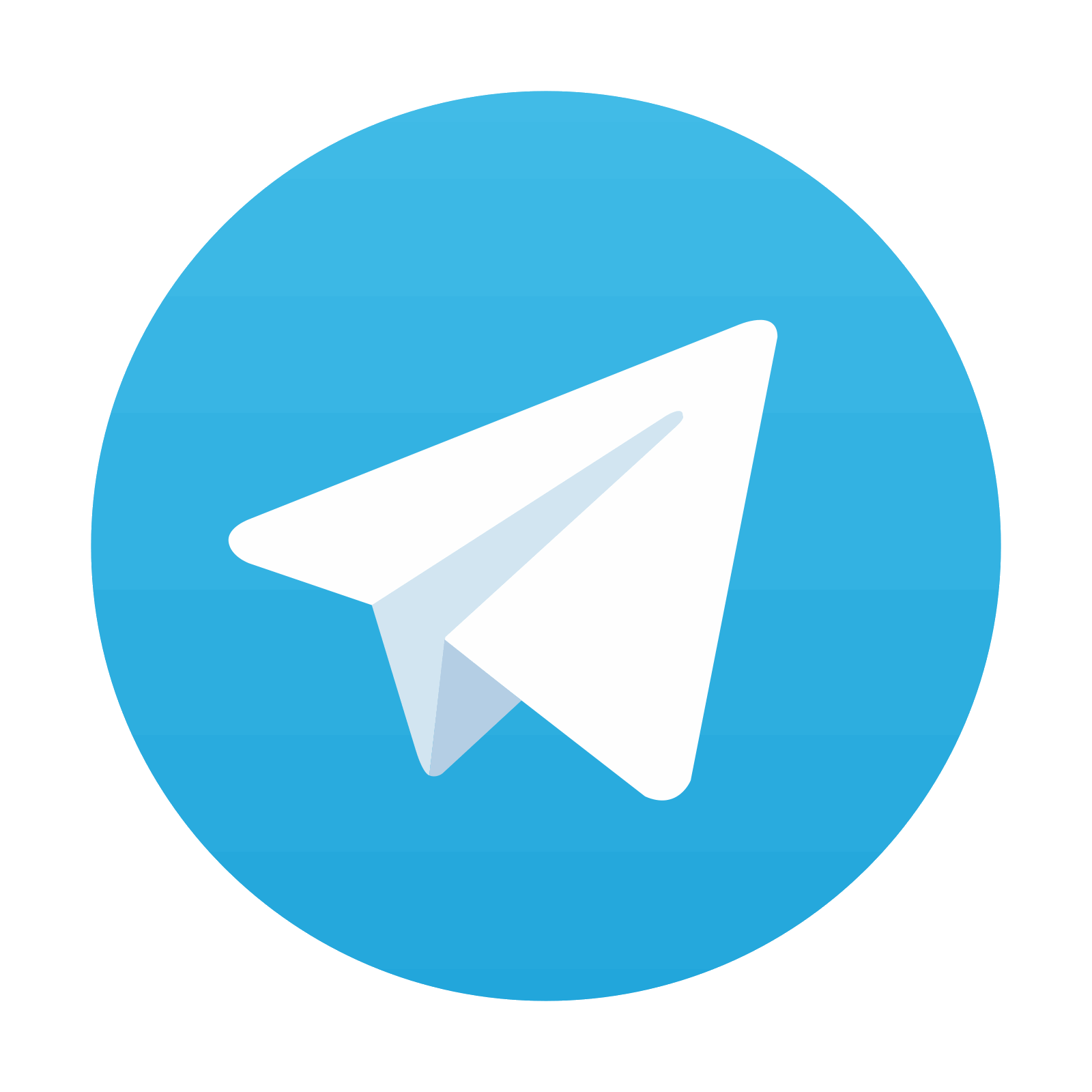
Stay updated, free articles. Join our Telegram channel
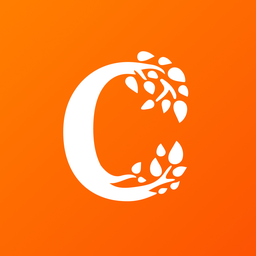
Full access? Get Clinical Tree
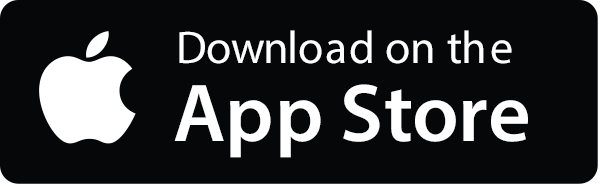
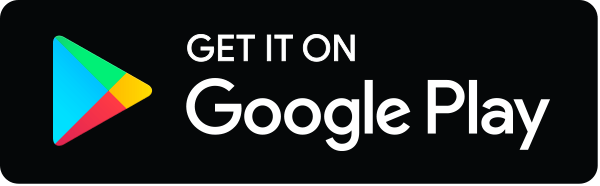