and Ashutosh Dash2
(1)
Nuclear Security and Isotope Division, Oak Ridge National Laboratory, OAK RIDGE, USA
(2)
Isotope Production and Applications Division, Bhabha Atomic Research Centre, Mumbai, India
3.1 Introduction
Alpha radionuclide therapy has a history starting with the anticipation of medical uses of radium which led to establishing several commercial facilities in the USA for large-scale production of radium (Metzenbaum 1905; Schales 1978; Macklis 1993). In 1913 Frederick Proescher published the first paper which described injection of radium for therapy of various diseases (Proescher 1913). The realization that failing health of both Pierre and Marie Curie had resulted from radiation exposure received during unprotected work with radioactive materials and the failure of early attempts to use naturally occurring radioactive materials for systemic therapy led to a major setback for the field of radionuclide therapy. Although use of radium sources for brachytherapy of cancer continued for a short time, this technology was replaced with other radioisotopes.
Over the years, systemic alpha radionuclide therapy remained essentially dormant, with the treatment of the inflammatory disease ankylosing spondylitis with 224Ra as one exception (Bertrand et al. 1978). Although this application is apparently no longer practiced, renewed interest in the use of alpha-emitting radioisotopes was later exemplified by studies with both 212Bi, the decay product of 212Pb, and artificially produced 211At (Zalutsky and Vaidyanathan 2000; Sgouros et al. 1998). Many other investigators then initiated studies on the development of radiopharmaceuticals using both naturally occurring and artificially produced alpha emitters (Brechbiel 2007; Nicolini and Mazzi 1999; Mulford et al. 2005). Most of the radioisotopes selected for investigation belong to one of the radioactive series of uranium, thorium, actinium, or artificially produced neptunium series (232U and 233U decay chains). Intermediate radioisotopes in the decay chains which have suitable physical half-life values for therapy have been selected for development of target specific radiopharmaceuticals, which include 225Ac, 212Bi, 213Bi, 227Th, and several other key examples which are subsequently discussed. A major challenge for use of alpha-emitting radioisotopes is that most decay to radioactive daughter products, which requires containing the decay products within the target. In the last decades, 213Bi emerged as the first alpha-emitting radioisotope to be used in modern human clinical trials in 1997 (Jurcic et al. 2002), and in more recent years, 223Ra constitutes the first systemic targeted alpha emitter approved by regulatory agencies for human use (vide infra).
The development and clinical use of Xofigo® (223RaCl3, originally designated as Alpharadin®) is discussed in detail in Chap. 12 and represents the first alpha therapy agent which has completed Phase I/II/III clinical trials and has been subsequently approved by the US Food and Drug Administration (FDA) and regulatory bodies in other countries for the routine treatment of metastatic bone pain in hormone-resistant prostate cancer patients (Parker et al. 2013).
3.2 Alpha Particles
Alpha particles are emitted by the decay of heavy radionuclides and are doubly charged energetic particles consisting of two protons and two neutrons (Fig. 3.1). After energy loss during travel through the medium, adsorption of two electrons then forms the helium nuclei. In a similar fashion, alpha particles can be produced by stripping two electrons from helium atoms and acceleration to high energy. The latter process is used to generate a beam of high-energy alpha particles in particle accelerators such as use of a cyclotron. The energies of the alpha particles obtained from the decay of radionuclides are in the range of 2–10 MeV, whereas alpha particles of up to a few hundreds of million electron volts (MeV) can be obtained by using an appropriately designed particle accelerator.
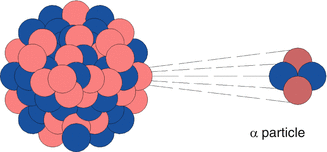
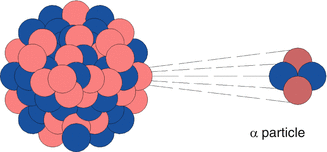
Fig. 3.1
Representation of the emission of an alpha particle via decay of a heavy radionuclide
3.2.1 Energy Dissipation of Alpha Particles in a Medium
Energy is lost from alpha particles as they pass through a medium resulting from a series of primary collisions with the negatively charged electrons of the atoms in the medium. These interactions result in ionization and excitation of the atoms in the medium. The energy lost by the moving alpha particles increases with de-acceleration. The loss of energy of a typical alpha particle in air is shown in Fig. 3.2, which is called the Bragg curve.
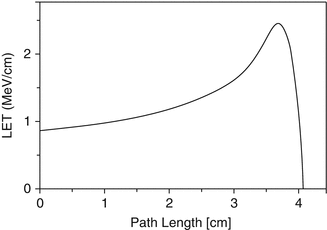
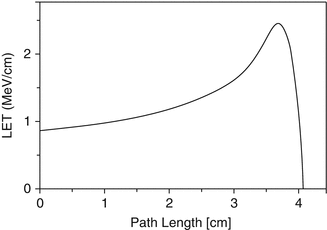
Fig. 3.2
Bragg curve representing the loss of energy and path length of an alpha particle in air
3.2.2 Linear Energy Transfer (LET)
The linear energy transfer (LET) is the measure of the energy transferred to the medium through which ionizing radiation passes. The LET term is used to quantify the effect of ionizing radiation within the medium such as a biological specimen. LET is expressed in energy lost per unit distance travelled (i.e., MeV/cm, Fig. 3.2). For practical purposes, LET of alpha particles is expressed in the more realistic keV/μm unit. The energy lost is directly related to the density of the medium traversed by the alpha particle. The alpha particles lose energy within a short distance of travel through aqueous medium (soft tissue), for example, usually in a few centimeters in air (Fig. 3.2). Biological systems have much higher density as compared to air. In biological soft tissue, for example, the complete energy of an alpha particle is dissipated within a very short path length, which is often less than 100 μm (i.e., only a few cell diameters). Figure 3.3 illustrates the estimated path lengths of two alpha particles with different energies in a biological system.
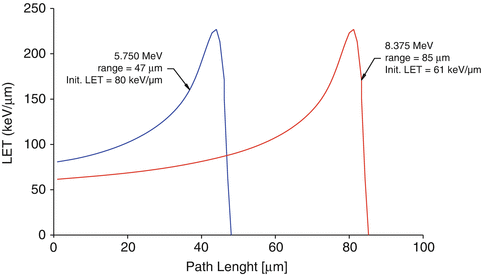
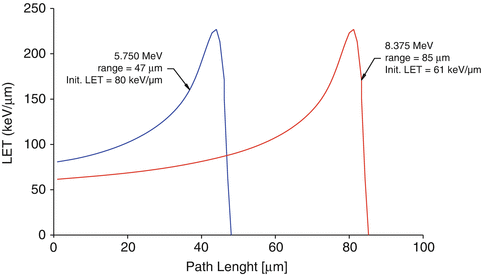
Fig. 3.3
Linear energy transfer (LET) of two different alpha particles in a biological system
The alpha particle LET is inversely related to energy, where higher energy alpha particles lose less energy within a given path length or they have lower LET and vice versa. For example, the LET of 5.750 MeV alpha particles is ~80 keV/m, whereas the path length is ~47 μm. The LET of 8.375 MeV alpha particle is ~60 keV/μm which have a path length of ~85 μm (12–15). The majority of the alpha particle energy loss occurs at the end of the path and at that moment the LET of the alpha particle increases to 200–250 keV/μm. All alpha particles irrespective of the initial energy will have identical LET at endpoint. Hence, the LET of alpha particles of different energies are quoted as the initial loss of energy per unit volume. As the naturally occurring alpha particles have energy values in the 4–8 MeV range, the average range of alpha particles in soft tissues is ~50–80 μm. A comparison between the short path length of energy deposition from alpha particle decay and β− decay is illustrated in Fig. 12.1. The advantages of high-LET radiation over low-LET radiation are the favorable dose distribution between tumor cells and normal cells and less dependence on tumor hypoxia, dose rate, and cell cycle position (Barendsen et al. 1966; Pouget and Mather 2001; Wang et al 2009). At the same time, the biological effects in the cells are more severe after high-LET radiation, resulting in high RBE.
3.2.3 Relative Biological Effectiveness (RBE)
The biological damage caused by ionizing radiations is expressed as the relative biological effectiveness (RBE), which relates to the biological damage of a radiation of interest compared with a reference radiation, usually 250 keV X-rays or the gamma rays emitted by 60Co (1.33 and 1.17 MeV). RBE is used as a multiplicative term to adjust the estimated absorbed dose so that it reflects the likelihood or severity of a biological effect. If the biological endpoint is stochastic such as cancer induction, then the RBE is approximately 20. In targeted therapy the relevant biological endpoint is not carcinogenesis but rather efficacy or toxicity. Such therapeutic endpoints are deterministic and the measure associated with them is not probability of occurrence (i.e., risk) but severity of toxicity or level of response. The RBE for such endpoints is in the range of 3–7. Among nuclear radiations, the RBE of alpha particles is the highest followed by β-particles and gamma rays. Owing to the high concentrated dose deposited along the track and short range in tissues on the order of cellular dimensions, alpha particles have a high probability of inducing damage to DNA, making them quite cytotoxic. By contrast radiation-induced cellular inactivation for low-LET radiation requires the accumulation of sublethal damage, achieved only at much higher dose. This specific radiation quality of alpha particles, characterized by localized spatial distribution of the imparted energy and high density of ionization per unit path length, causes direct DNA damage rather than indirect free radical-mediated DNA damage. Toxicity originates from the increased frequency of clustered DNA double-strand breaks observed with high-LET radiation (Azure et al. 1994; Goodhead 1994; Howell et al. 1997). The higher the RBE value, the greater the damages encountered from radiation for the same absorbed energy (Azure et al. 1994; Schwarz et al. 1998; Zalutsky and Narula 1988) (Fig. 3.4).
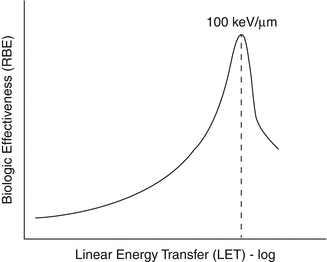
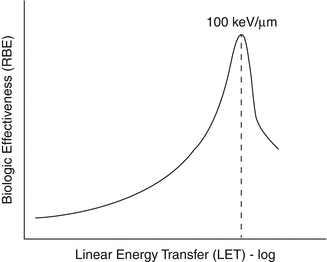
Fig. 3.4
RBE values as a function LET illustrating the much higher RBE values for alpha-emitting radioisotopes
The cytotoxicity of α particles has been shown as independent of both dose rate and oxygenation status of the cells (Hall 1994). In addition to directly damaging DNA, alpha particles have been demonstrated to cause chromosomal instability even in the descendants of non-irradiated stem cells in the vicinity of irradiated cells. This “bystander effect” reflects the potential for interaction between irradiated and non-irradiated cells in the production of genetic damage (Lorimore et al. 1998). The spatial distribution of alpha-particle sources and the geometry of the cells (thickness, diameter of the cell nucleus, distribution of DNA) have to be considered as important parameters in order to correlate the absorbed dose distribution with the observed biological response for the tissue of interest (tumor and/or critical organs).
3.2.4 Interaction of Alpha Particles in a Biological System
The dimensions and size of human cells are quite variable; however, the average cell diameter of most common cells is the order of 10–100 μm. Hence, most alpha particles lose their complete energy within 2–10 cell diameters. Since the total energy of alpha particle is dissipated in a small volume of cells, the damage caused to biological systems by an alpha particle-emitting radionuclide decaying in the vicinity of human cells is very high. In radiation biology, the effect of radiation is measured by multiplying the absorbed dose with a term called the quality factor (QF) or radiation weighing factor (W R). The W R of the alpha particles is 20. Hence, alpha-emitting radionuclides cause very high radiation damage once they are inside a living system. As per the International Atomic Energy Agency (IAEA) classification, alpha-emitting radionuclides are listed in Group 1, which means they have the highest toxicity and hence the maximum permissible body burden (MPBB) is the lowest (IAEA 1963).
3.2.5 Basis of Alpha Radionuclide Therapy
Alpha particles cause primary and secondary ionizations to atoms with which they interact and create several ions and excited molecules within cells. The net result of this intracellular turbulence is intermolecular bond biomolecule cleavage. Such events can occur with cytoplasm and all intracellular components as well as the cell nucleus. The desirable feature of alpha radiotherapy is the ability of the decaying alpha particle to cause intramolecular DNA bond cleavage within targeted cancer cells. The DNA molecule is double stranded and cleavage can occur in one or both the strands. The cartoon in Fig. 3.5 depicts the traverse of an alpha particle through a double-stranded DNA molecule and the resultant DNA fragments.
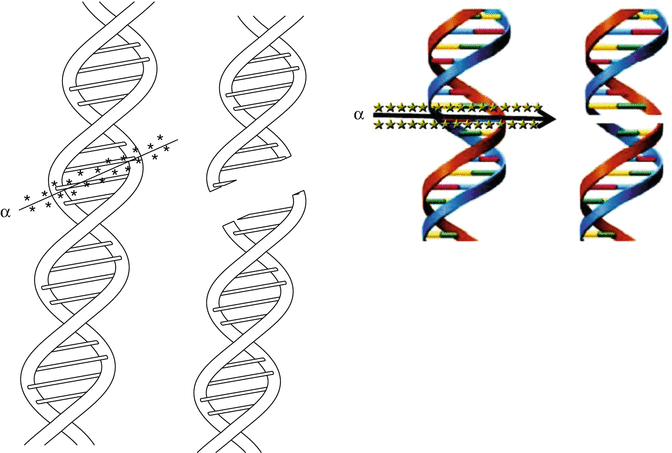
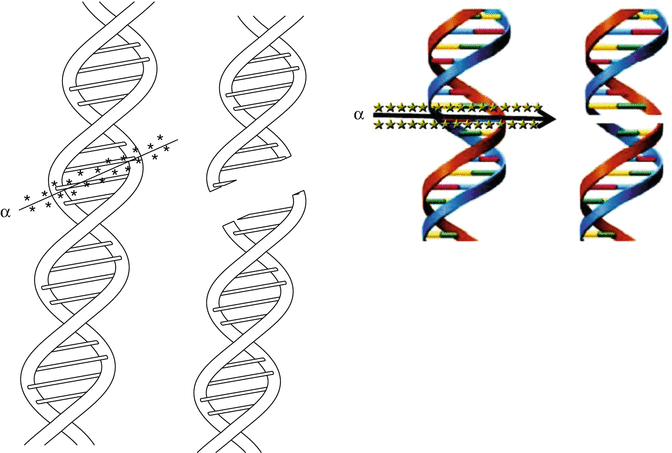
Fig. 3.5
Cartoon showing the passage of an alpha particle through the double-stranded DNA molecule and the resultant double-strand break fragments
The DNA molecule has inherent ability to repair single-strand damage; however, the repair of a scission in double-stranded DNA to its original form is generally not possible. This damage is the intended therapeutic effect to sterilize cells which can then no longer divide. Repair of double-stranded scission of the DNA molecule can lead to gene mutation, which is an undesirable yet very uncommon outcome of controlled radiation exposure. There is an estimated 20–40 % probability that an α particle interacting with the DNA molecule can ensure breakage of the double strand leading to eventual cell death. In contrast, − radionuclide therapy requires several β− decays (“hits”) to take place in the vicinity of the cell to ensure cell killing (Wessels and Rogus 1984; Humm and Cobb 1990). However, alpha decay must occur in close proximity to the target cell because of the very short path length. In β− radionuclide therapy, the much longer soft tissue penetration (Fig. 12.1) always irradiation of many cells in addition to the targeted cell because of the “cross-fire” effect to induce nontarget cell death. For this reason, an essential condition for ensuring α therapy efficacy is the high degree of accuracy required to deliver the radiation dose to the target cells and at the same time to spare adjacent healthy cells. In the same context, β− emitting radionuclides are generally more desirable for therapy of solid tumors.
3.2.6 Dosimetry
For α-particle emitters, accurate dosimetry calculations are required. In light of the perceived need to estimate the dose distribution, detailed knowledge of the activity distribution as a function of time at the cellular and subcellular levels as well as an accurate representation of the geometry is a necessity (Sgouros et al. 2011; Chouin and Bardies 2011). Efforts to use clinical scintigraphic imaging turned out to be futile owing to the requirement of spatial resolution of the order of several mm, which is two orders of magnitude greater than the microscopic scale at which one dose is deposited with alpha particles (Allen 2011). Owing to the important stochastic variations in the energy deposited in cell nuclei, macroscopic dosimetry approaches become less relevant and microscopic approaches are required. In this premise, the fundamental quantities required for microdosimetry are specific energy (energy per unit mass) and linear energy (energy per unit path length through the target) which can be used for the calculation adopting analytical methods (convolution via Fourier transforms) or Monte Carlo simulation methods (Bardiès 2000). In this context of long half-lives, the distribution of both the parent and all daughters must take into account while performing dosimetry calculations (Koch et al 1999). Absorbed dose calculations for alpha-particle emitters can be routinely performed using the dosimetry formalism described in the Medical Internal Radiation Dose (MIRD) Committee Pamphlet 21 (Bolch et al. 2009) with the guidance provided in MIRD Pamphlet 22 (Sgouros et al. 2010).
3.3 Alpha-Particle-Emitting Radionuclides for Radiotherapy
There are over 100 radionuclides categorized in the Chart of Nuclides which decay by α particle emission, of which only a few are practical for radionuclide therapeutic applications. The main reasons for this restricted number of alpha emitters which are investigated include the inability for production in adequate activity levels for therapy and/or unfavorable physical half-lives. Table 3.1 summarizes the physical characteristics of a number of alpha-emitting radionuclides which are of interest for RT. With the exception of artificially produced 211At and 149Tb, all others are emitted from the decay chains of long-lived actinide elements. Most of these radioisotopes of interest are present in radioactive equilibria with their long-lived radioactive parents. For example, the decay product of the thorium series (Fig. 3.6) contains alpha-particle-emitting 224Ra (t 1/2 3.66 days) and 212Bi (t 1/2 60.55 min), which are both of great current interest for radionuclide therapy. Since the parent and daughter in each case are in secular equilibrium (Chap. 7), their respective activity levels will be equal to the activities of the long-lived parent radionuclides.
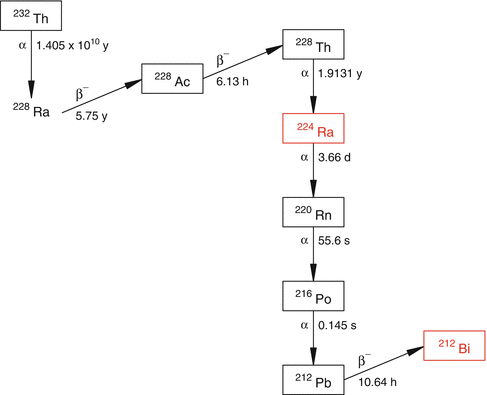
Table 3.1
Examples of key alpha-emitting radionuclides useful for alpha radionuclide therapy
Radionuclide | Half-life | Daughter | aEnergy of particles (keV) | Energy of β− particles | Energy of rays, KeV |
---|---|---|---|---|---|
Actinium-225 | 10.0 days | 221Fr | 5935 (100 %) | 86 keV | |
Astatine-211 | 7.21 h | 207Bi 211Po | 5870 (41.7 %) 7450 (58.2 %) | 786 keV (58.2 %) | |
Bismuth-213 | 45.59 min | 213Po | 5869 (93 %) | 1427 (64.5 %) 986 (30.4 %) | 440 (26.1 %) |
Bismuth-212 | 60.55 min | 208Tl (35.94 %) 212Po (64.06) | 6089 (27.1 %) 6050 (69.9 %) | 2254 (55.4 %) | |
Radium-223 | 11.435 days | 219Fr | 6559 (100 %) | Nil | 269 (13.7) |
Radium-224 | 3.66 days | 220Rn | 5685 (100 %) | Nil | 241 (4.1 %) |
Terbium-149 | 4.118 h | 145Eu (17 %) 149Gd (83 %) | 3967 (17 %) | 2432 (31.5 %) | 853 (15.45 %) 817 (11.6 %) |
Thorium-227 | 18.72 days | 223Ra | 6038 (100 %) | Nil | 256 (7 %) 236 (11.5 %) |
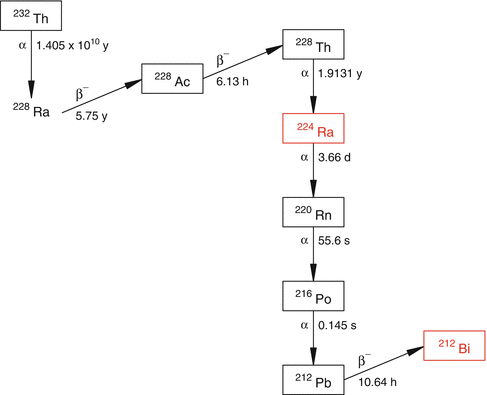
Fig. 3.6
The decay chain of the naturally occurring thorium (232Th) series. The therapeutic radionuclides 224Ra and 212Bi are part of the 232Th series
Alpha radionuclide therapy is generally preferred for special applications, which include situations targeting isolated cancer cells circulating in the lymphatic and vascular systems, the regression of metastatic cancer cell clusters, and for disrupting the vasculature of solid tumors. Radionuclides of current or potential use for alpha radionuclide therapy and short summaries of preclinical and clinical studies are discussed in the following sections of this chapter.
3.3.1 Astatine-211
Astatine-211 is an artificially produced alpha radionuclide with a half-life of 7.2 h and which decays via a branched pathway to stable lead as shown in Fig. 3.7. Electron capture (EC) decay (58.3 %) of 211At leads to the production of 211Po (t 1/2 0.516 s) which decays by emission of an alpha particle (7.45 MeV, 100 %) to stable 207Pb. The second branch (41.7 %) decays to 207Bi via emission of an alpha particle (5.87 MeV, 100 %). Although long lived (t 1/2 33.4 years), the 207Bi will not contribute to any radiation dose during therapy. Through these two branching routes, each of the decaying 211At atoms contributes to the production of one alpha particle per decay. In addition, decay of 211At emits β−particles (58.3 %, βmax 786 keV). The soft tissue ranges of the two alpha particles are ~55 μm and ~80 μm with a mean initial LET of ~100 keV/μm (Fig. 3.8). The electron capture decay of 211At to 211Po (58 %) emits 77–92 KeV K X-rays of polonium which are suitable for scintigraphic imaging.
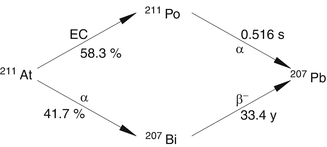
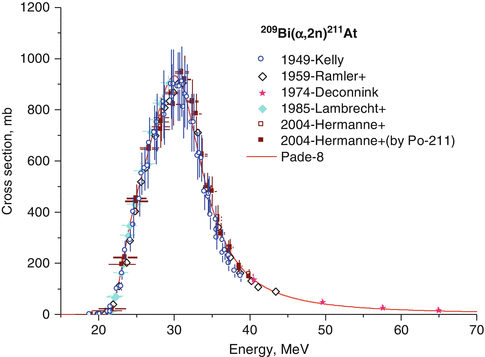
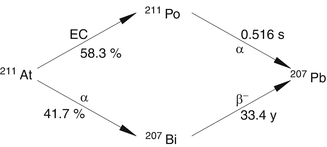
Fig. 3.7
Nuclear decay scheme of 211At showing the two branched chains leading to its decay to 207Pb
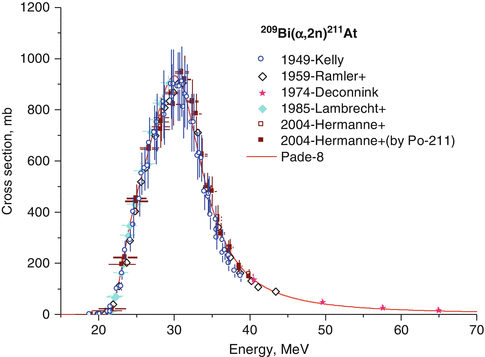
Fig. 3.8
Excitation function of 209Bi(α,2n)211At
3.3.1.1 Production of 211At
Astatine-211 is produced by alpha-particle bombardment on 209Bi targets by the 209Bi(α,2n)211At reaction (IAEA 2009; Schwarz et al. 1998; Henriksen et al. 2001; Lebeda et al. 2005). Bismuth is mononuclidic and 209Bi is present in 100 % abundance. The nuclear reaction cross section approaches a value of one barn at 28 MeV (Fig. 3.8). The thick target yield of 211At is reported to be between 30 and 40 mCi/μA.h at 28–29.5 MeV (IAEA 2009). For this reason high activity levels of 211At can be produced by use of a suitable solid target for irradiation in cyclotrons having α beams of 30 MeV and a beam current in the range of a few hundred μA. Dry distillation at 650 °C releases volatile 211At, which can be removed by use of a carrier gas. The distilled 211At is trapped in polyether ether ketone (PEEK) tubing maintained at −77 °C with a mixture of ethanol and ice. The trapped 211At is then recovered by using organic solvents (Zalutsky and Pruszynski 2011).
Astatine-210 will also be coproduced during production of 211At through the competing 209Bi(α,3n)210At reaction. The decay product of 210At, 210Po, has a half-life of 138 days, decays by alpha emission, and localizes in the bone marrow. Hence, 210At is an undesirable radionuclidic impurity. The cross section for the production of 210At is practically absent below 30 MeV (Fig. 3.9), and hence the beam energy is maintained below 30 MeV during target bombardment.
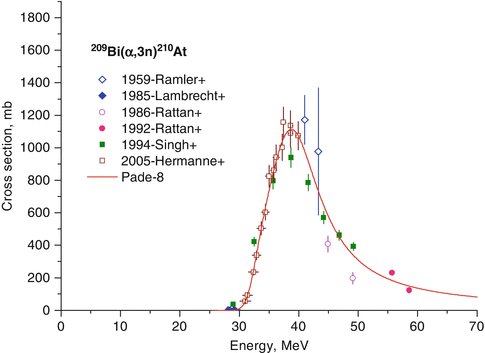
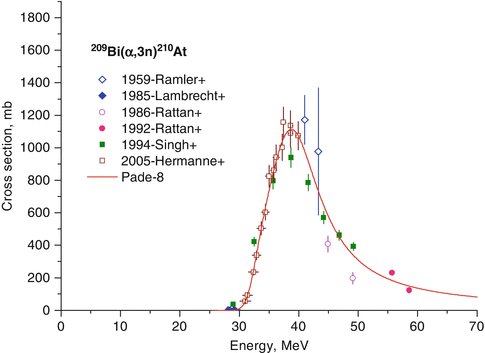
Fig. 3.9
Excitation function of 209Bi(α,3n)210At
3.3.1.2 Astatine Chemistry
Astatine is the last member of the halogen family and hence the least electronegative in the series and thus exhibits some metallic properties. Astatine does not exist in any stable isotopic form, and unfortunately, the chemistry of At cannot be elucidated by chemical synthesis and characterization of nonradioactive compounds in the usual manner. The carbon–halogen bond strength decreases in higher homologues, and hence the carbon–astatine bond is the weakest, resulting in vulnerability of astatine compounds to deastatination. Astatine-211-labeled aromatic compounds are usually synthesized by exchange halogenation or by electrophilic substitution on aromatic rings. Low specific activity is a serious limitation with exchange labeling. Electrophilic substitution of 211At to the phenolic ring has been demonstrated; however, the carbon–astatine bonds formed with such activated aryl rings are labile, leading to poor in vivo stability of the products. Electrophilic astatodemetallation of organometallic derivatives, preferably organostannanes, is used for preparation of stable 211At tracers (Fig. 3.10).


Fig. 3.10
Electrophilic astatodemetallation of organostannanes
Utilizing the metallic properties of astatine, radiolabeling of antibodies using DTPA complex of 211At has also been reported (Ning et al. 1998). The anionic character of astatine has been used to attach the 211At anion to soft metal cations such as mercury, rhodium, and iridium complexed with bifunctional chelating agents. Since it is a halogen anion, any free astatide (211At−) has a tendency to be localized in the thyroid, spleen, bone marrow, stomach, and lungs (Visser et al. 1981). Hence, 211At-labeled radiopharmaceuticals must exhibit high chemical stability in vivo, to avoid unwanted radiation dose to the above organs, especially to the thyroid.
3.3.1.3 Astatine-211 Radiopharmaceuticals
Astatine-211 has been extensively evaluated for alpha RT therapy in animal models especially and a comprehensive description of this work is described in a review article (Vaidyanathan and Zalutsky 2008). A brief description of preclinical studies with the various 211At-labeled agents follows.
Astatide (211At−)
Like iodide, astatide (At−) is taken up by the thyroid tissue mediated through the sodium iodide symporter (NIS), which is a membrane protein expressed on the basolateral surface of the thyroid epithelial cells. For this reason 211At− could potentially be used for the treatment of cancers of thyroid origin; however, the consequences of targeting to sites such as the thyroid would have to be evaluated. The potential of NIS-mediated uptake of 131I for non-thyroid cancer through gene incorporation has been proposed which could also be extended to astatine. The short half-life of 211At as compared to 131I is an advantage since the efflux of activity from the tumor is generally rapid and does not match the long half-life of 131I (t 1/2 8.02 days). An evaluation of the long-term effect of [211At]astatide in NIS-induced tumor-bearing mice showed complete irradiation of the tumor in 3 months (Petrich et al. 2006; Brown and Carpenter 1991; Lundh et al. 2006; Carlin et al. 2003).
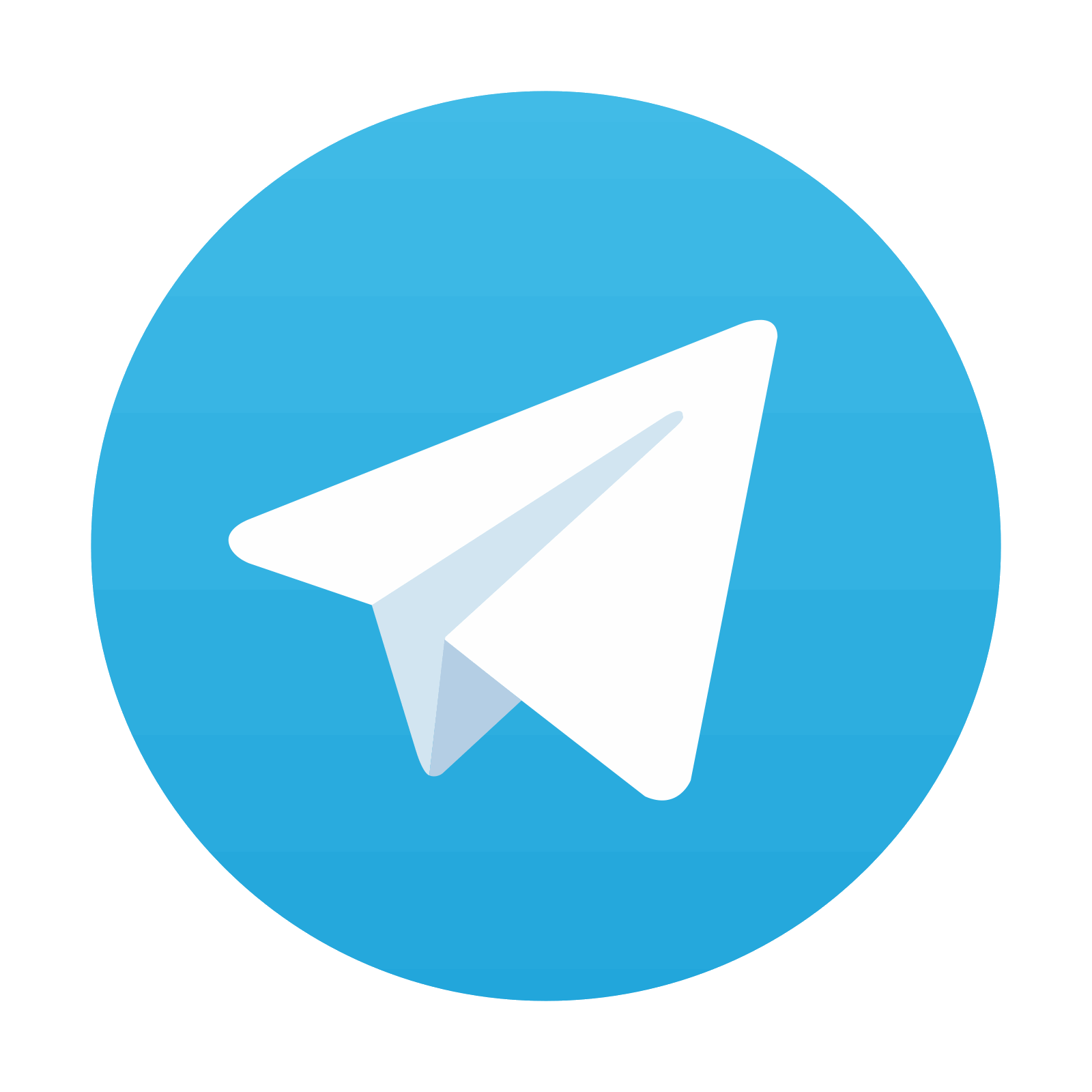
Stay updated, free articles. Join our Telegram channel
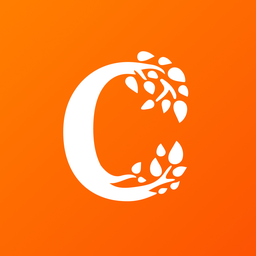
Full access? Get Clinical Tree
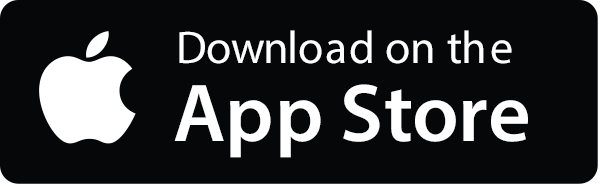
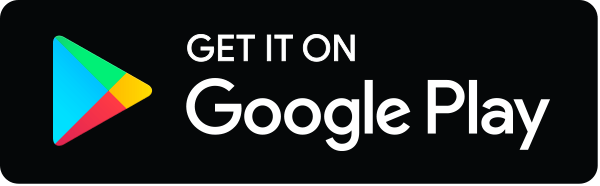
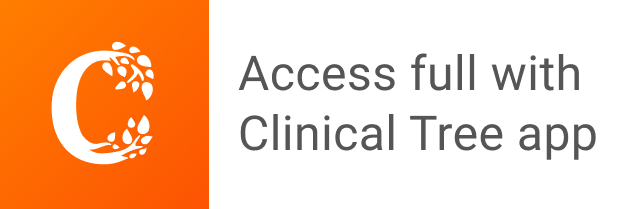