Fig. 48.1
Ligand-gated ion channels are probably the most relevant targets for general anaesthetics. A dark green or pink spot indicates significant potentiation or inhibition, respectively, of agonist actions at the receptor by the anaesthetic with an EC50 or IC50 that is no greater than three times higher than the EC50 for producing immobility. A light green or light pink spot indicates little potentiation or inhibition, respectively, at concentrations that were less than three times the EC50 for immobility; an empty spot indicates no effect at any concentration tested (From Rudolph and Antkowiak 2004 used with permission)
Two less commonly used intravenous anaesthetic agents, that have not yet been mentioned, are ketamine and dexmedetomidine. Ketamine causes potent analgesia and when used as the sole hypnotic, it causes a dissociated state (in which the patient may appear conscious but is dissociated from the environment and applied stimuli). Both effects are mediated via an antagonist effect at the NMDA glutamate receptor (Franks 2008; Alkire et al. 2008b). Dexmedetomidine also has some weaker analgesic effects and at low doses causes arousable sedation not dissimilar to a natural sleep state, with general anaesthesia occurring at much higher doses. These effects are mediated by an agonist effect at α2 adrenergic receptors, with sedative and hypnotic effects probably mediated by actions on these receptors in the locus coeruleus.
For the volatile anaesthetic agents, agonist effects at the GABAA receptor appear to be important (Alkire et al. 2008b) but with binding of the agents to different binding sites than those for propofol and the barbiturates. The molecular mechanism of action of the volatile agents may also include significant potentiating effects at two-pore potassium and glycine channels and inhibitory effects at NMDA receptors (Franks 2008).
48.8 Early PET Studies of the Global and Regional Changes in Cerebral Glucose Metabolism Caused by Anaesthetic Agents
The earliest PET studies of anaesthesia were performed in rats, in which 18FDG was used during propofol anaesthesia, and showed dose-dependent global reductions in cerebral glucose utilization (Ori et al. 1986; Dam et al. 1990; Cavazzuti et al. 1991). These studies led Dr Michael Alkire to perform the first PET studies of anaesthesia in humans. In the first, 18FDG was used before and during propofol anaesthesia, and as in the rat studies, it was found that anaesthesia caused reductions in glucose metabolism in all measured cortical and subcortical areas (Alkire et al. 1995). In this study, marked regional differences in tracer uptake were noted, with a greater suppression of cortical than subcortical activity. At this time, it was just becoming apparent that the molecular mechanism of action of propofol was mediated by the GABAA receptor. These regional differences even led Alkire and colleagues to speculate that differences in the regional distribution of GABAA receptors were responsible for the regional variations in metabolic activity.
Alkire and his group then went on to study the influence of isoflurane anaesthesia on glucose metabolism, again using the 18FDG tracer (Alkire et al. 1997). Although they found a similar degree of global reduction in metabolic activity to propofol, they found less regional variability with isoflurane. They found similar reductions in cortical and subcortical activity and widespread, fairly uniform reductions in activity across all cortical and subcortical areas. This widely quoted study was taken to provide support for a non-specific, physical explanation of the mechanism of action of the anaesthetic agents.
A subsequent study with an older volatile anaesthetic agent, halothane, showed similar global reductions in metabolic activity to isoflurane and propofol (40 %, vs 46 and 55 %, respectively) (Alkire et al. 1999). In general, the pattern of regional metabolic effects was similar to that found with isoflurane, with the exception of the cerebellum, where metabolism was more suppressed by halothane. As with isoflurane, midbrain metabolism was more suppressed than with propofol, and here the authors speculated that this is because the volatile anaesthetics have a strong influence on cholinergic transmission, whereas propofol does not.
Each of the above three studies involved only a small number of subjects, in whom anaesthetic dose was titrated to loss of consciousness, with concurrent EEG monitoring, using the Bispectral Index (BIS) monitor (Covidien, USA), a monitor of depth of anaesthesia, which gives an output of between 0 and 100, where zero indicates no brain electrical activity, 100 the completely awake state and values between 40 and 60 are taken to represent adequate anaesthesia. The authors combined the data to show that the degree of global reduction in glucose metabolism correlated strongly with the Bispectral Index (Alkire 1998).
Although the above findings were sometimes taken to provide evidence of a non-specific mechanism of anaesthetic activity, they also indicated differences between anaesthetic agents and regional variations in metabolic suppression, providing evidence against the theory of a single non-specific mechanism of action. In the subsequent years, the evidence against this theory continued to mount up, particularly as investigators designed more sophisticated studies, combined imaging modalities and compared the results of different drugs and modalities. PET methodology played an important role in this. Indeed, by 2005 there was sufficient evidence from studies involving PET measures of cerebral blood flow and metabolism with different anaesthetic agents for Alkire to perform a conjunction analysis. This analysis highlighted the considerable regional differences among different agents, thereby providing strong evidence for specific anaesthetic effects (Alkire and Miller 2005). Further, despite differences in molecular mechanisms, and in regional neurophysiological effects, all agents with anaesthetic effects had in common a significant effect on the thalamus (see Fig. 48.2). Based on this work, and on previous animal findings (Angel 1993), Alkire became a strong proponent of the ‘thalamocortical switch hypothesis’, the theory that thalamocortical resonant loops are essential for consciousness and that all anaesthetic agents interrupt consciousness by interrupting the thalamocortical interactions and interrupting onward transmission of ascending electrical signals (Alkire et al. 2000).
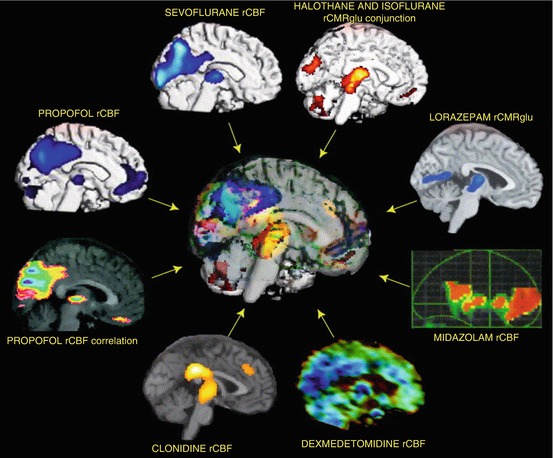
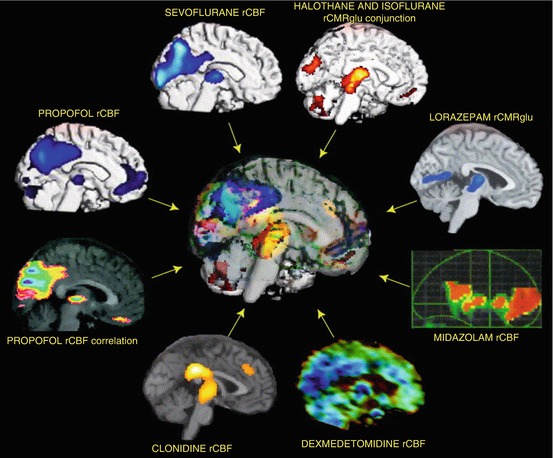
Fig. 48.2
The regional effects of anaesthetics on brain function in humans who were given various anaesthetic agents at doses that altered the level of consciousness. The data are from seven different groups of investigators and encompass the study of eight different agents. The regional effects were measured using blood flow- or glucose metabolism-based techniques. All images show regional decreases in activity caused by anaesthesia compared to the awake state, except the propofol correlation image, which shows increasing anaesthetic dose correlates with decreasing blood flow. The figure identifies that altered blood flow and metabolism in the thalamus is a common finding associated with anaesthetic-induced unconsciousness (From Alkire and Miller 2005 used with permission)
Subsequent work using PET has assessed various combinations of metabolism, blood flow, blood volume and receptor occupancy, for different drugs (sometimes in combination). To simplify the findings, they will be subdivided by drug type, starting with the intravenous agents (propofol, ketamine and the alpha2 agonists) and finishing with the inhaled agents (volatile anaesthetic agents, nitrous oxide, xenon).
48.9 PET and Propofol
The early work by Alkire et al. (1995) has already been mentioned. Although this showed global reductions in glucose metabolism, the effects of propofol on relative glucose metabolism were different for different areas (where regional metabolism as a proportion of global metabolism is considered). Thus, whereas the relative glucose metabolism decreased for most cortical areas and the thalamus, there were relative increases for the temporal lobe, hippocampus, basal ganglia, midbrain and cerebellum.
Fiset and colleagues used H2 15O PET to study changes in global and regional cerebral blood flow (rCBF) associated with mild sedation, deep sedation and unconsciousness induced by propofol (Fiset et al. 1999). Concordant with the findings of Alkire, they found dose-related decreases in global CBF. Further, they identified evidence supporting specific molecular effects of propofol. They found that propofol preferentially decreased rCBF in brain regions involved in arousal, autonomic functions and associative functions, such as the medial thalamus, midbrain, cuneus and precuneus, posterior cingulate cortex and orbitofrontal regions. Interestingly, there were strong correlations in rCBF in the midbrain and thalamus, indicating strong functional interactions between the arousal systems of these regions.
In a more sophisticated study, this group measured CBF responses (using H2 15O PET) to vibrotactile stimulation during graded sedation and anaesthesia (Bonhomme et al. 2001). In general, this study demonstrated that propofol caused dose-dependent decreases in rCBF in the thalamus, bilateral precuneus and bilateral posterior cingulate gyri. During unconsciousness, there were widespread increases in rCBF when PaCO2 was increased by 20 %, which can be explained by the fact that CO2 reactivity is preserved with propofol. In the awake state, vibrotactile stimulation produced a predicted pattern of rCBF changes – increases in relative rCBF in the left thalamus and primary somatosensory cortex (S1), left superior frontal gyrus and bilateral secondary somatosensory (S2) cortices. Most interesting was the finding that propofol produced dose-dependent, selective regional effects on the responses to vibrotactile stimulation. At low sedative doses, the S1 responses were abolished, and at moderate sedative doses the S2 responses were abolished (and accompanied by changes in sensory perception), whereas thalamic responses were only abolished when consciousness was lost.
Scheinin et al. in Finland used H2 15O PET to assess changes in absolute and relative CBF during graded general anaesthesia (from moderate to deep levels) with propofol and sevoflurane (Kaisti et al. 2002). Propofol caused a significant global decrease in absolute rCBF, but with a ceiling effect at the lowest anaesthetic concentration (target concentration 6 mcg/ml – approximately the EC50, the concentration required to suppress responses to pain in 50 %; mean measured concentration was 7.4 mcg/ml). The transition from the awake state to that at EC50 was associated with relative rCBF decreases in the thalamus, midbrain, cuneus, precuneus, posterior limbic system and parietal and frontal cortices. The lack of absolute changes in rCBF with propofol, even at 2 × EC50, suggested that flow-metabolism coupling is maintained during propofol anaesthesia. In a subsequent study by the same group, also involving propofol anaesthesia, but at a lower dose (mean measured concentration 3.7 mcg/ml), similar changes in rCBF, regional oxygen metabolism and regional cerebral blood volume (assessed by H2 15O, 15O2 and C15O tracers, respectively) were found (Kaisti et al. 2003). This provided evidence of intact flow-metabolism at this low dose, which suggests that low-dose propofol anaesthesia does not produce direct vasodilatory effects. Another group administered a similar level of propofol anaesthesia (targeting a BIS of 35–40) to volunteers and found similarly matched changes in flow and metabolism (in this case metabolism was assessed with 18FDG PET) (Schlunzen et al. 2012).
Most studies of the influence of propofol metabolism have assessed glucose or oxygen metabolism. One study, however, has assessed regional cerebral protein synthesis rates using the tracer L-[1-11C] leucine (Bishu et al. 2009). No effect of propofol on protein synthesis was found (measured propofol concentrations were in the range 4.2–8.1 mcg/ml).
The remaining studies to be discussed in this section were focused on receptor effects of propofol. Alkire and Haier performed a study in which they correlated their findings concerning regional cerebral glucose metabolism rates under propofol and isoflurane anaesthesia, with data previously published by other investigators on the regional distributions of different neurotransmitter systems (Alkire and Haier 2001). The latter data were acquired from post-mortem immunochemistry investigations (Braestrup et al. 1977; Zezula et al. 1988; Enna and Snyder 1977). They were able to show that there were significant correlations between regional reductions in glucose metabolism during propofol anaesthesia and the regional distribution of benzodiazepine binding sites (from studies using 3H diazepam which binds to both neuronal and mitochondrial benzodiazepine receptors and 3H flunitrazepam which binds only to neuronal benzodiazepine binding sites on GABAA receptors). There were also weaker correlations with the distribution of opioid binding sites, but no correlations with distribution densities of adrenergic, muscarinic and serotonin binding sites.
Salmi and colleagues later showed that propofol increases 11C-flumazenil binding, indicating that propofol enhances the affinity of GABA for GABAA receptors (Salmi et al. 2004). At around the same time, another group found that propofol anaesthesia was associated with a reduction in muscarinic receptor binding by the tracer [N–11C-methyl]-benztropine (Xie et al. 2004), but this finding was difficult to interpret. Although it may have indicated binding of propofol to muscarinic receptors, it could also have been the result of a change of affinity of the receptor for benztropine or an increase in benztropine metabolism caused by propofol. Several years later, members of the same group performed an intriguing study in which they measured CBF with a H2 15O technique at baseline, after loss of consciousness with propofol and administration of the cholinesterase inhibitor, physostigmine (Xie et al. 2011). Some, but not all, volunteers regained consciousness after physostigmine administration, despite constant blood and brain concentrations of propofol. The authors found that loss of consciousness was associated with decreases in rCBF in the thalamus and precuneus and that among those that regained consciousness after physostigmine, restoration of consciousness was associated with increases in rCBF in these same structures. It is assumed that the increased ACh levels caused by physostigmine result in activation of cholinergic pathways and an increase in thalamic throughput of electrical signals sufficient to cause a return of consciousness.
Taken together, these studies indicate that propofol causes a specific pattern of changes in regional metabolic activity and CBF, but with a key effect at the thalamus, and that loss of consciousness is associated with interruption of thalamocortical signalling. Finally, the work from the past decade and a half supports the hypothesis that propofol acts at specific protein targets, most notably at the GABAA receptor.
48.10 PET and Ketamine
Ketamine is an older anaesthetic agent than propofol but is seldom used alone for inducing or maintaining surgical anaesthesia because of a propensity to cause troublesome psychiatric adverse effects. It remains interesting clinically and is being used with increasing frequency as an adjunct during propofol anaesthesia. Known and potential beneficial effects include improved cardiovascular stability, neuroprotection, reduced acute post-operative pain, potent antidepressant activity and attenuation of post-operative hyperalgesia and chronic pain.
Ketamine is also of scientific interest because it acts as an antagonist at the NMDA receptor and causes different neurophysiological changes to the other hypnotics. When used alone it causes impaired consciousness, but with cortical electrical activation accompanied by increases in glucose metabolism (Vollenweider et al. 1997) and rCBF. The reasons behind these effects are not clear. The drug antagonizes an excitatory pathway, but presumably this results in activation of other excitatory pathways. Impaired consciousness is probably the result of impaired connectivity or communication between regions of the brain important for consciousness.
Langsjo and colleagues studied the effects of sub-anaesthetic doses of racemic ketamine on rCBF, regional oxygen metabolism (rCMRO2) and regional cerebral blood volume (rCBV) using the PET tracers H2 15O, 15O2 and C 15O (Langsjo et al. 2003). Ketamine caused global dose-dependent changes in absolute rCBF, with the biggest changes in the anterior cingulate, insula and frontal cortex (areas involved in pain processing) and also in the thalamus and putamen. As expected mean systemic arterial pressures were increased. There were no significant changes in rCMRO2, and hence the oxygen extraction fraction was reduced. rCBV was only increased in the frontal cortex. Taken together these findings were consistent with known behavioural and electrophysiological changes seen with ketamine sedation but did give rise to a suggestion of altered flow-metabolism coupling. The authors performed a follow-up study, this time using 18FDG to assess regional changes in glucose metabolism (rCMRG) associated with sub-sedative racemic ketamine doses (at the highest dose used in the previous study) (Langsjo et al. 2004). Region of interest analyses showed global absolute increases in rCMRG, but with the greatest changes in thalamus, frontal and parietal cortex. Voxel-based analyses showed increases in relative rCMRG in frontal, temporal and parietal cortices. These changes occurred in a similar distribution and magnitude to the rCBF findings of the previous study (Langsjo et al. 2003), suggesting maintenance of flow-metabolism coupling. The authors suggested that the absence of rCMRO2 changes was the result of non-oxidative glucose metabolism in response to ketamine-induced glutamate release.
The same group then studied the effects of s-ketamine on cerebral blood flow and metabolism, using a complex study design (Langsjo et al. 2005). H2 15O and 15O2 scans were performed during one session, at three moments: awake, during low-dose sub-sedative s-ketamine administration and during much higher dose s-ketamine anaesthesia. 18FDG scans were performed 3 weeks before the main study and finally also towards the end of the s-ketamine anaesthesia. The authors found dose-dependent increases in total CBF and in absolute rCBF in almost all regions studied. During low-dose s-ketamine administration, the greatest increases were in the anterior cingulate, whereas at anaesthetic doses the largest increase (86.5 %) was in the insula. At low doses there were no significant increases in rCMRO2, whereas at anaesthetic doses rCMRO2 was increased only in the frontal cortex (by 15.7 %). During anaesthesia rCMRG was only increased in the thalamus, and CBV was increased >50 %. These findings show that CBF is increased in excess of metabolic needs, suggesting a disturbance of flow-metabolism coupling. While the excess flow may be perceived to be protective, these findings confirmed the long-held views of anaesthesiologists (based on the work of others using different methodologies) that ketamine is not a suitable agent for use during neurosurgery, where the increases in CBF and CBV can cause increased brain volume, increased intracranial pressure and impaired surgical access.
Finally, the same group studied the influence of sub-anaesthetic doses of ketamine on 11C-flumazenil binding (Salmi et al. 2005). They showed no interference in binding, thereby confirming that ketamine has minimal effects on the GABAA receptor.
48.11 PET and Alpha2 Agonists
PET technology has only been used to study the alpha2 agonists a few times. Clonidine and dexmedetomidine are the two most commonly available alpha2 agents in clinical use. Although clonidine has been available for quite a long time, it has mainly been used intravenously for its antihypertensive effects. More recently it has been used as a preoperative oral sedative premedication and perioperatively as an intravenous analgesic adjunct. Dexmedetomidine has been in use just over 10 years in the USA. Initially it was mostly used for ICU sedation; more recently it has been used as a sedative during surgical procedures such as awake craniotomy. It was first registered in Europe in late 2011.
Prielipp used H2 15O scans to assess the changes in rCBF during and after low and high sedative dose infusions of dexmedetomidine. The agent caused marked reductions in global CBF and also reductions in absolute rCBF in 13 out of 14 regions of interest studied. There were no significant differences in patterns of rCBF between low and high sedative doses. Despite the rapid pharmacokinetics of dexmedetomidine, CBF remained decreased when assessed 30 mins after the end of the dexmedetomidine infusion (Prielipp et al. 2002).
Another group studied the influence of clonidine infusions on rCBF (assessed by H2 15O PET), clinical status and EEG (Bonhomme et al. 2008). Clonidine caused rousable sedation and on the EEG spindle patterns similar to those found during non-rapid eye movement sleep. There was a negative correlation between measured plasma clonidine concentration and rCBF in a network of regions including the thalamus, prefrontal cortex, orbital and parietal association cortex, posterior cingulate cortex and precuneus. Changes in these regions are associated with natural sleep. These regions also form part of the default mode network, whose component areas show strong correlations in activity (assessed by fMRI) during the resting state, but not during task performance conditions (Mason et al. 2007; Stamatakis et al. 2010).
48.12 PET and the Volatile Anaesthetic Agents
Volatile anaesthetic agents have been the subject of many PET studies. These have mainly focused on the neurophysiological changes in flow and metabolism and have shown generally concordant findings, with only minor variations between studies, possibly related to differences in dose. Minor variations were found according to drug, despite some chemical differences between the agents studied, such as structure, molecular weight, degree of halogenations, water and lipid solubility, etc. Halothane is a halogenated hydrocarbon that is now seldom used in the first world. Isoflurane, sevoflurane and desflurane are halogenated ethers. The latter two agents are the most commonly used inhalational anaesthetic agents, although there are few PET studies involving desflurane.
The early work by Alkire, showing that isoflurane anaesthesia caused a 46 % reduction in rCMRG with fairly uniform regional distribution, has already been mentioned (Alkire et al. 1997). Alkire then studied the effects of halothane on rCMRG, since this agent had been shown in earlier studies to cause less uniform rCMRG reductions (Alkire et al. 1999). Halothane anaesthesia was found to cause a 40 % reduction in global rCMRG; but in keeping with the prior animal work, there were some regional differences, with the greater metabolic reductions being found in the basal forebrain, thalamus, limbic system, cerebellum and occipital cortex.
In the above studies, halothane and isoflurane were administered until an end point of loss of consciousness (the mean concentrations administered were 30 and 60 % less than the concentrations required to prevent a response to a surgical incision – the so-called MAC value, a measure of potency). Kaisti studied the effects of surgical levels of sevoflurane anaesthesia – at concentrations that were 1.0, 1.5 and 2.0 multiples of the MAC value – on rCBF using H2 15O (Kaisti et al. 2002). At 1.0 MAC sevoflurane caused global decreases in absolute rCBF (regional decreases were between 36 and 53 % of baseline). Increasing doses caused a redistribution of flow, with increases in rCBF in thalamus and cerebellum, suggesting a disturbance of flow-metabolism coupling.
In a follow-up study, using H2 15O, 15O2 and C 15O to study rCBF, rCMRO2 and CBV, respectively, Kaisti titrated sevoflurane and propofol anaesthesia (with and without N2O) to a moderate depth of anaesthesia, guided by the BIS (Kaisti et al. 2003). Sevoflurane concentrations were thus lower than in the previous study – 0.75xMAC without N2O and 0.6 MAC with 70 % N2O. Sevoflurane without N2O reduced the total CBF, but only caused rCBF decreases in some areas (occipital cortex, cerebellum, caudate and thalamus). The most significant changes in relative rCBF were in the thalamus, cuneus, frontoparietal cortex and cerebellum. Addition of N2O caused a global return to baseline rCBF values, with the most significant relative rCBF increases in the occipital cortex and pons. With regard to CMRO2, sevoflurane caused a global reduction, with absolute decreases in rCMRO2 in all areas studied. The greatest reductions in relative rCMRO2 were in the thalamus, cuneus, frontoparietal cortex and cerebellum. Addition of N2O increased rCMRO2 in the parieto-occipital region and cerebellum, but not quite to baseline/awake levels. At the doses studied, sevoflurane with and without N2O did not alter rCBV. It was associated with a tendency towards reduced oxygen extraction fraction, more marked in the presence of N2O, suggesting that metabolism was more suppressed than flow, suggesting a degree of disturbed flow-metabolism coupling.
The above findings were generally confirmed by Schlunzen et al., although some increases in rCBF were found at lower doses (Schlunzen et al. 2004). The study involved use of H2 15O to study changes in rCBF associated with sub-anaesthetic through to anaesthetic doses of sevoflurane (0.2, 0.35 and 1.0 MAC multiples). At all doses, sevoflurane increased rCBF in the anterior cingulate and decreased rCBF in the cerebellum. Compared with the baseline state, 0.2 MAC sevoflurane decreased rCBF in the inferior temporal cortex and lingual gyrus. 0.35 MAC increased rCBF in the middle temporal cortex and lingual gyrus, while decreasing rCBF in the thalamus. The latter finding is unsurprising given that some volunteers displayed no response to a verbal stimulus, whereas other showed only sluggish responses. Finally, compared with 0.35MAC, 1MAC increased rCBF in the insula and decreased rCBF in the posterior cingulated, lingual gyrus, precuneus and frontal cortex. Similar patterns of changes were found in subsequent study, using similar methodology and equipotent isoflurane doses (Schlunzen et al. 2006).
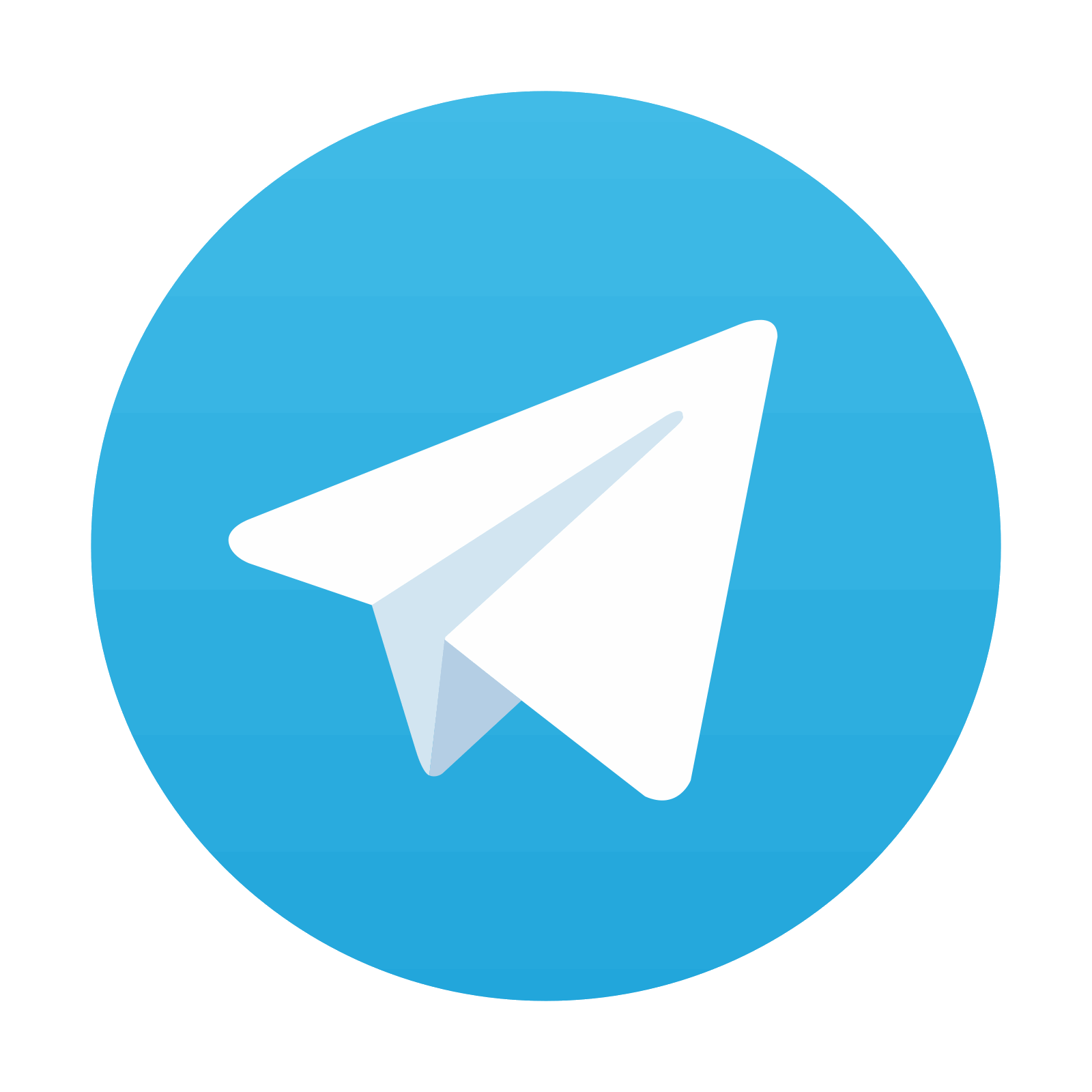
Stay updated, free articles. Join our Telegram channel
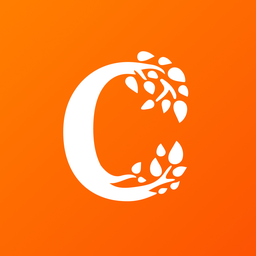
Full access? Get Clinical Tree
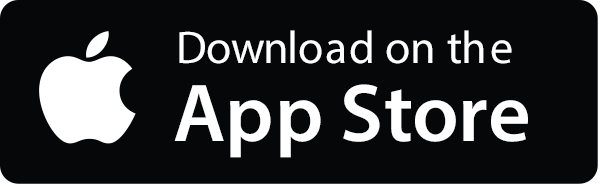
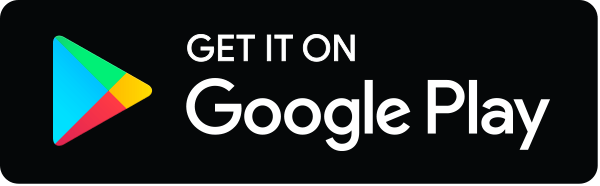